Fig. 1.
Examples of behavioural measures used to identify motor dysfunctions after injury to the motor cortex. (a) Cylinder test. (b) Skilled reaching test. (c) Forepaw inhibition in swimming. (d) Sunflower seed opening. (e) Tongue extension. See text for details.
Motor cortex injury provides a useful technique not only for understanding the functions of the motor cortex but also for studying the effectiveness of rehabilitative treatments in stimulating functional recovery. We shall outline the current methods of injuring motor cortex and techniques of studying the evolution of functional outcomes after the injury.
1.3 Motor Cortex Stimulation
The first documented use of a functional approach using electrical stimulation was carried out by Fritsch and Hitzig (17). They stimulated the cortical surface of dog brains with short DC discharges from a battery and reported five areas that gave rise to different types of movements. A few years later, Ferrier reported his experiments with dogs, cats, rabbits, guinea pigs and later with monkeys where he used seconds of continuous alternating current to induce movements (18, 19). Ferrier is credited as describing “purposive movements” and the first somatically arranged motor map. These two sets of researchers argued over the “correct” method of stimulation. Ferrier took the position that shorter trains of stimulation did not allow complex movements whereas Hitzig countered that longer stimulation trains evoked seizures (20). During the later part of the nineteenth century, Beevor and Horsley (21) working with monkeys used both short trains to elicit a muscle twitch and long trains to create a map of purposeful movements. They argued that both approaches were valuable ways to view cortical organization. While their later work was primarily based on short trains they also demonstrated that careful removal of the gray matter and stimulation of the remaining descending white matter tracts produced the same map of the body as did short train stimulation of the cortex itself. These influential researchers appear to have set the research field on a course typified by the view that the function of the motor cortex was defined by the simple activation of the descending connections to the spinal cord.
Sherrington made many important insights into the function of motor cortex by stimulating the surface of the motor cortex with short bursts of electrical stimulation at the lowest intensity possible that allowed the observation of a movement. He examined the relation between cortical points by stimulating one site and observing the movement evoked by stimulation at another site. He noted that prior stimulation of one site could alter the movement at another site. Even though he was using short bursts of stimulation he reached the important conclusion that the map was not anatomically fixed but plastic and dependent on its activation history. That is to say the motor cortex is the repository of a large variety of sequences of movements (22). Several researchers still using cortical surface stimulation with short trains in primates, including humans, detailed multiple cortical maps (23–27). However, the “problem” with surface stimulation is that it requires relatively high intensity stimulation currents to elicit movements. Thus according to the view that larger currents lead to more current spread and deriving a map should not involve overlapping areas of excitation, the technique of surface stimulation leads to a relatively low resolution motor map. In order to stimulate with smaller current intensities and derive the highest resolution motor map possible, Asanuma pioneered the intracortical microstimulation technique for deriving movement representations.
In the late 1960s and early 1970s, Hiroshi Asanuma and colleagues worked in the motor cortex of cats (28, 29) and capuchin monkeys (30). They inserted fine insulated microelectrodes with an exposed tip into different layers of the motor neocortex and passed a minimal amount of cathodal (negative charge) current with short pulse-widths (0.2 ms) and short trains (40 ms trains of 13 pulses at 300 Hz) to elicit a muscle or joint movement. On average their current intensities were 1/100th the intensity of those used to evoke movement on the cortical surface. These “standard” short train methodologies have been successfully used for decades and have generated a substantial literature on the functioning of motor cortex. Hall and Lindholm (31) were the first to adopt the short train ICMS technique to work in rats. In the 2000s, Michael Graziano reinvigorated the use of long-train intracortical microstimulation methodologies. Using stimulation trains an order of magnitude longer than traditional ICMS (500 ms versus <50 ms), he evoked complex, coordinated movements involving multiple joints in monkeys (32, 33). The train duration used was similar to both the timescale of forelimb reaches and motor cortex neuron activity during movement in monkeys (34). It appears that train duration is a critical factor mediating evoked movements by ICMS. At behaviourally relevant time scales, complex movements similar to spontaneous behaviour are evoked. As train duration is reduced, the movements become truncated, eventually leading to individual joint flexions. Long-train ICMS has more recently been successfully applied in the rat (35).
ICMS provides a valuable tool in the study of both the functional organization and sensitivity of cortical movement representations in addition to the nature and property of cortical-evoked movements (36). Below we provide a detailed description of the use of short-train stimulation techniques in both rats and mice and long-train stimulation techniques in rats.
2 Studying the Effects of Motor Cortex Injury
2.1 Inducing Injury
The motor cortex can be damaged selectively in a wide variety of ways, each of which requires slightly different equipment (Table 1). In most procedures a craniotomy is performed and the motor cortex is removed or damaged by gentle aspiration, electrocoagulation with a metal electrode, or stripping away of the fine surface blood vessels to produce ischemia (pial stripping). Other less focal procedures include either temporary or permanent occlusion of the middle cerebral artery. The cortex can also be damaged by injecting drugs that produce vasoconstriction such as endothelin-1 (ET-1). A final procedure is to give an i.v. injection of a photosensitive dye, Rose Bengal. When the skull of Rose-Bengal treated rats is illuminated with a focused beam of light for 10 min, cortical thrombosis is induced in the underlying tissue, at least partly due to the induction of apoptosis. The advantage of Rose Bengal is that no craniotomy is necessary.
Table 1
Equipment required for making selective motor cortex lesions
Procedure | Specialized equipment |
---|---|
Suction | Aspiration pump, tubing and glass pipette |
Electrocoagulation | Stainless steel jor platinum electrode, current generator |
Pial stripping | Sterile cotton swab |
MCA occlusion | Either vessel clamps (transient) or electrocautery machine (chronic) |
Endothelin-1 | Injection syringe and infusion pump |
Rose Bengal | Fiber optic light system to deliver focused light |
In those procedures using a craniotomy, the skull is exposed and the size of the desired motor injury is marked on the skull. The bone is thinned with a high speed drill and then the remaining bone is removed with fine rongeurs. The size of the opening will vary with the region of interest (see maps below). For aspiration, electrocoagulation, and pial stripping, the dura is then cut with a pointed (#11) scalpel blade and removed. We have found it best to also cut through the grey matter along the edges of the craniotomy in order to make it easier to delineate the size of the injury. The aspiration procedure involves using gentle suction and removing only the grey matter within the delineated region. For pial stripping, the cotton ball is dipped in sterile saline and then used to gently wipe off the surface blood vessels (e.g., (37)). Electrocoagulation is performed by delivering a 1-mA anodal current through an uninsulated stainless steel or platinum electrode during repeated (15 s each) traverses through the exposed cortex for a total of 2 min (e.g., (38)).
There are several methods for the application of ET-1. These include the topical application across the surface of the cortex in the region of the craniotomy, multiple injections of the ET-1 into the grey and/or white matter, and injection of ET-1 adjacent to the middle cerebral artery. Dosages vary considerably across laboratories and require pilot work but, in general, the higher the dose, the larger the injury (see details in (39)).
Occlusion of the middle cerebral artery (MCA) can be done at different points on the MCA beginning at the point it courses upward from the base of the brain, which produces significant cortical and subcortical injury, or at any point along the MCA as it spreads out over the cortex (e.g., (40)). The more the focal injuries of the cortex are seen, the more distal the occlusion is performed. A permanent occlusion is made by using electrocoagulation of the artery whereas transient occlusions are made by placing clips on the artery for 10–20 min and then removing the clips. The former procedure mimics a permanent blockade of the MCA, such as with a large emboli, whereas the latter procedure mimics transient attacks such as in vasospasm.
For the Rose Bengal procedure, the photochemical Rose Bengal is infused into the femoral vein via a microinjection pump within 2 min (20 mg/kg). A light source is then positioned close to the skull, focussed on the desired area of cortex and turned on for 10 min. If the light is not cold (fiber optic), then the skull surface temperature must be monitored and the skull kept cool by cool air flow (e.g., (41)).
2.2 Measuring Behavioural Changes
There are many techniques to measure motor functions (see (12, 42) for extensive discussions). The choice of behavioural measures varies with the questions being addressed, however. In particular, studies in which the focus is on the details of behavioural changes in order to infer functions of the motor cortex typically use more sophisticated measures (see (12)) than studies looking for treatments to stimulate functional recovery. Most of our work has been in the latter category and we have developed a test battery to allow us to measure both forelimb and oral/facial functions (see Fig. 1). These measures are sometimes supplemented with measures of hindlimb coordination as rats traverse either a narrow beam (10) or a horizontal ladder (16). Similar measures can be used for mice, although the apparatus must be scaled down.
We have been surprised to discover that all of the lesion techniques produce strikingly similar deficits with the severity varying mostly with the extent of injury rather than the etiology (37, 39). One important difference, however, is that the compensatory changes in the cortex vary depending upon the lesion etiology (37, 43). The simplest way to study this is to examine dendritic length and spine density using Golgi-type analyses of neuronal morphology (e.g., (37, 38)). For example, whereas the pial stripping stimulated dendritic growth in pyramidal cells in layer V of the forelimb region of the undamaged hemisphere, suction ablation did not. In contrast, suction increased spine density in the pyramidal neurons in the anterior cingulate cortex but pial stripping did not. Such differences could be important in designing rehabilitation procedures.
2.3 Cylinder Test (Forepaw Asymmetry)
Forelimb use for weight support during explorative activity is examined by placing individual rats in a transparent cylinder 20 cm in diameter and 30 cm high for 3 min (44). A mirror is placed underneath the cylinder at an angle to allow the observer to videotape the animal’s activity from a ventral point of view. Forelimb use is measured during vertical exploration. Each forepaw contact with cylinder wall is counted. An asymmetry score of forelimb use in wall exploration is calculated for each animal [(affected forelimb)/(affected + unaffected)] to obtain a score where 0.5 represents perfect symmetry and any number closer to zero would suggest a decrease in the use of the affected limb.
2.4 Skilled Reaching
Training boxes for reaching are made of clear Plexiglas with the dimensions 26 cm high, 28 cm deep and 19 cm wide. The fronts of boxes are constructed of 2 mm bars separated from each other by a 9-mm gap. Animals are trained to reach for food fragments weighing ∼30 mg each. Reaching success is calculated by dividing the number of successful reaches (food grasped and eaten) by the total number of reaches. It takes about 15 days for animals to reach a consistent baseline, which is about 60–70% for rats and 40–50% for mice.
2.5 Sunflower Seed Opening
The ability of animals to successfully open and consume seeds using their limb and digits is measured following procedures of Whishaw et al. (45). The animals are given experience with sunflower seeds for several days before testing. Five sunflower seeds are placed in a corner of a Plexiglas box (45 cm × 14 cm × 35 cm) that the animals have been accustomed to eating the seeds in. Their behaviour was video recorded on the fifth day. Two measures used to quantify the behaviour are videotaped and two measures recorded (1) time: the total amount of time spent on manipulating, opening the shell and consuming the seeds and (2) the number of pieces of shells after animals retrieve and eat the seeds. If the animal shredded the shell into pieces too small to count, a maximum of 30 pieces of shell is assigned.
2.6 Swimming Task
Rats are trained for several days to swim to a visible platform located at one end of a rectangular aquarium (120 cm × 43 cm × 50 cm) filled with warm water. During training, the animals are released from the opposite end of the pool and are given practice until they learn to swim directly to the platform without touching the pool walls. Normal rats and mice hold their forepaws immobile under their chins while swimming and use their hind limbs to propel through the water. Animals are videotaped on three swims and the number of forelimb strokes with either paw is counted. Normal rats typically make no strokes whereas normal mice are more variable and usually make some strokes.
2.7 Tongue Extension
This task requires animals to stick out their tongue to lick palatable food, which has been spread on a ruler (15). Animals are placed into the reaching boxes with the food tray removed. We have had good success with both peanut butter and a slurry of warm water and chocolate chip cookie. Normal rats can extend their tongues up to 12 mm whereas mice can reach about 3 mm.
3 Standard “Short Train” Intracortical Microstimulation Methods
3.1 Equipment
Table 2 lists the equipment necessary to perform ICMS in rats and mice.
Table 2
Equipment required for intracortical microstimulation experiments
Rodent stereotaxic apparatus |
Microscope with camera connected to a computer |
Computer with imaging software |
Stimulator with monophasic and biphasic stimulation capability |
Microdrive |
Servo-controlled warming blanket |
Electrode puller and beveller if using glass electrodes |
Drill and fine surgical tools |
Disposables (liquid silicone to protect brain surface) |
Video camera and movement analysis software |
Amplifiers and filters for EEG and EMG analysis |
3.2 Electrodes
Insulated electrodes made from either glass or tungsten are typically used. The more traditional glass electrodes are created by heating and pulling borosilicate glass capillaries on a micropipette puller. The advantage of this system is that the electrodes can be custom made to have specific tip sizes, angles and impedances. The glass electrodes are filled with between 3.0 and 3.5 M NaCl and normally have impedance values in the 1–1.5 MΩ range. Tungsten electrodes are commercially available and can be reused for extended periods of time.
3.3 Anesthesia
Mapping at the correct anesthetic depth is probably the biggest “trick” to deriving an excellent map. This is because the anesthesia itself likely has the largest influence on the amount of current needed to induce a movement. For ethical reasons, the animal must be sufficiently anesthetized to prevent pain and yet if the anesthetic level is too deep then it may not respond to ICMS at all. It is critically important to revisit responsive points over the course of mapping the cortex and re-derive some of them to determine if the anesthesia level is fairly constant. A few physiological indices that can also be used as feedback indicating anesthesia level are breathing rate and vibrissae whisking as well as a foot reflex (in rats) or a tail reflex (in mice) in response to a gentle pinch (3, 46). Recording EEG and examining the amount of power at several frequencies in real time is also recommended to ensure that anesthesia levels are fairly constant (47).
Deriving motor maps requires an anesthetic agent that has the following general characteristics (1) it maintains muscle tone so that stimulation-induced movements can be detected, (2) it has metabolic kinetics that give rise to a fairly constant anesthetic depth over several hours, (3) it allows a balance between excitation and inhibition so that reasonable levels of stimulation current intensities can be applied directly to layer V, and (4) it has a wide therapeutic window making it difficult to inadvertently kill the animal.
For rats, researchers commonly use a combination of the NMDA receptor antagonist ketamine and the alpha-2 adrenergic agonist xylazine. Ketamine provides anesthesia and some analgesia while xylazine provides sedation, anesthesia and a little muscle relaxation. Rats initially receive an intraperitoneal (i.p.) injection of ketamine (100 mg/kg) and xylazine (5 mg/kg). Supplemental injections are required with either ketamine alone (25 mg/kg), or a cocktail of both ketamine (17 mg/kg) and xylazine (2 mg/kg) and are delivered i.p. as required throughout the surgical procedure to maintain a relatively constant level of anaesthesia. It is critically important to keep careful and detailed notes on the amount of drugs given to the animals and the length of the surgical procedure because the quantity of anesthetic needs to match between control and experimental conditions. Again, this is because the depth of anesthesia influences the amount of current used to determine a movement threshold.
Mice will succumb if they are given the same dosage of ketamine and xylazine as rats. We have had success with an initial i.p. injection of ketamine (20 mg/kg) and xylazine (1 mg/kg). Supplemental injections of ketamine alone (5 mg/kg), or a cocktail of both ketamine (3.4 mg/kg) and xylazine (0.4 mg/kg) should be delivered i.p. as required throughout the surgical procedure to maintain a constant level of anaesthesia.
3.4 Craniotomy
Using a drill a craniotomy is performed by removing the skull and exposing the sensorimotor neocortex of one hemisphere. In rats this window extends approximately 4 mm anterior and 3 mm posterior from bregma and from midline to 5 mm lateral of midline. In mice, the window extends approximately 4 mm anterior and 3 mm posterior from bregma and from midline to 3 mm lateral of midline – yes, mice have proportionately bigger maps than rats. These windows allow for a complete mapping of the forelimb area and the window can be extended if the researchers are interested in probing for other body movements. The brain will often swell following the craniotomy. In order to reduce the pressure caused by edema, an 18-gauge needle is used to make a small puncture in the cisterna magna. Dura is then carefully removed from the cortical surface and a silicone fluid at body temperature is used to cover the neocortical surface to keep it from desiccating.
3.5 Stimulation Grid and Interpenetration Distance
A digital image of the exposed portion of the brain should be captured and displayed on a computer (Fig. 2). This image can then be overlaid with a grid. The grid directs the location of the electrode penetration points at the intersections of the grid lines and at a central point in the middle of each square except when a blood vessel is present at a penetration point, in which case, the point is not derived.
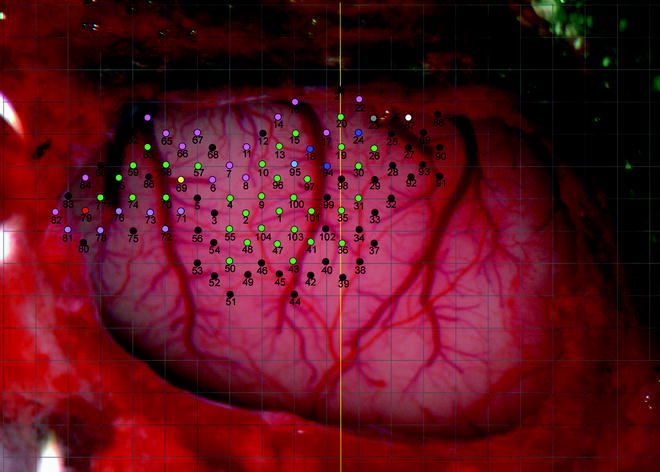
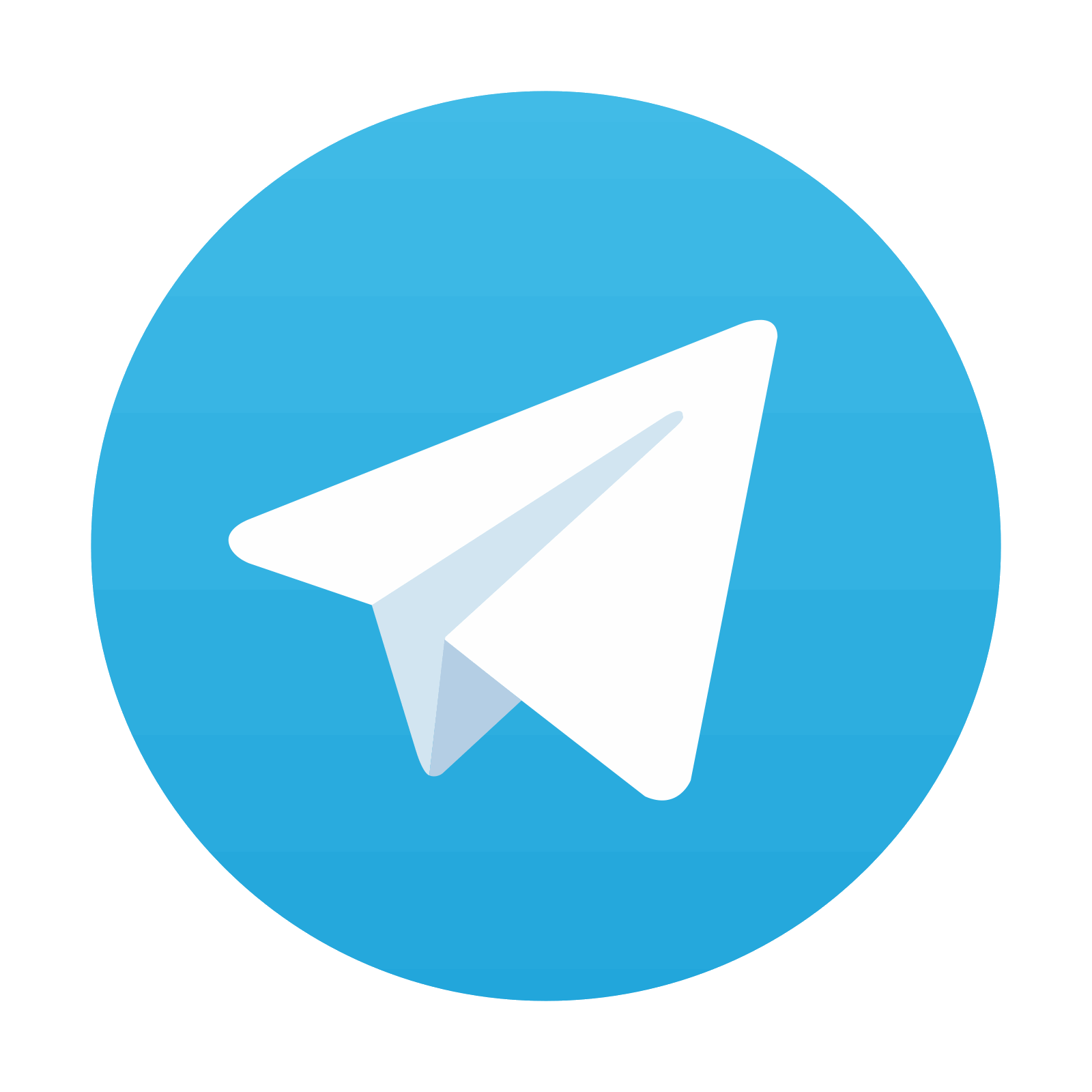
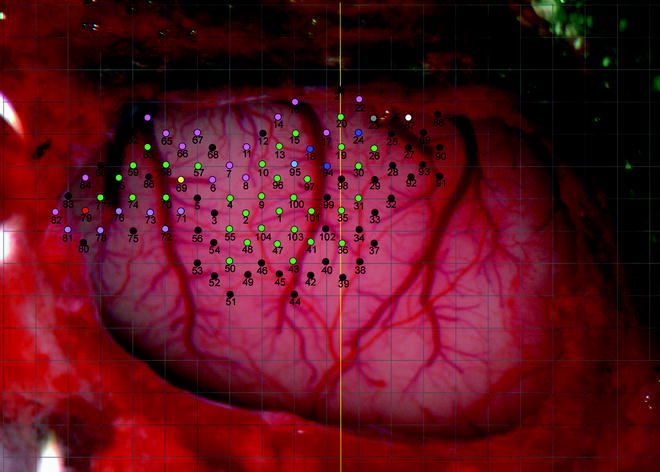
Fig. 2.
Photographic image of part of the surface of the rat left hemisphere with skull and dura removed. Pial vessels are clearly shown. A 500 by 500 μm grid (grey lines) is overlaid on the image. Coloured circles represent penetration points of the electrode (black = non-responsive, green = wrist, red = digit, dark blue = elbow, light blue = shoulder, pink = whisker, purple = jaw, yellow = neck, grey = trunk, white = hindlimb). The numbers represent the order in which the points were derived. The vertical yellow line indicates bregma. Left is anterior, right is posterior, top is medial, bottom is lateral.
< div class='tao-gold-member'>
Only gold members can continue reading. Log In or Register a > to continue
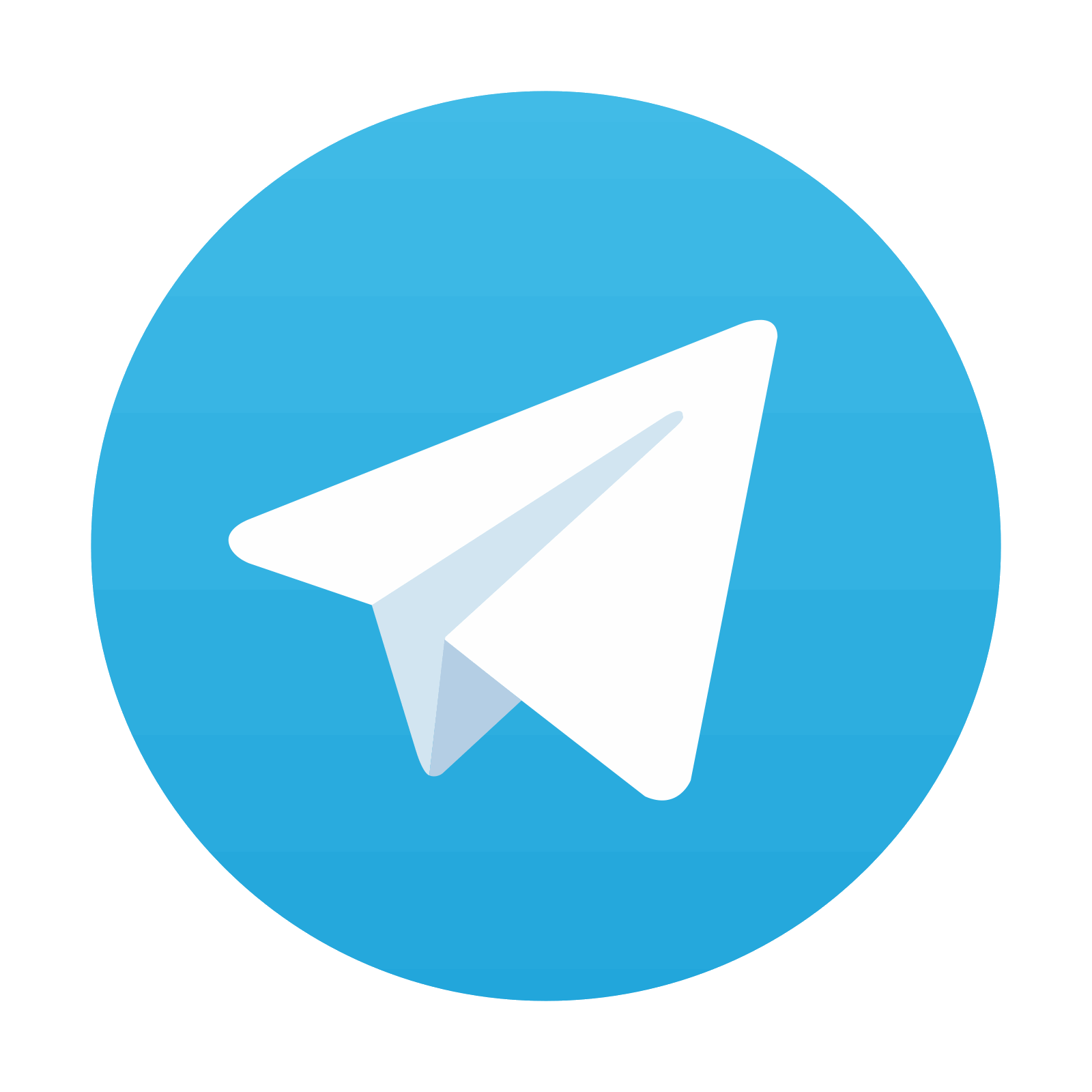
Stay updated, free articles. Join our Telegram channel
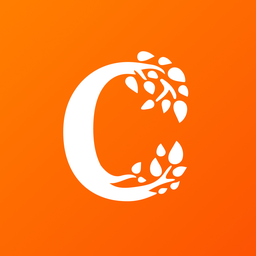
Full access? Get Clinical Tree
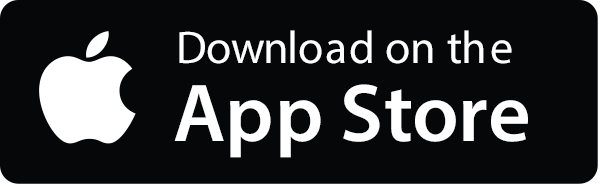
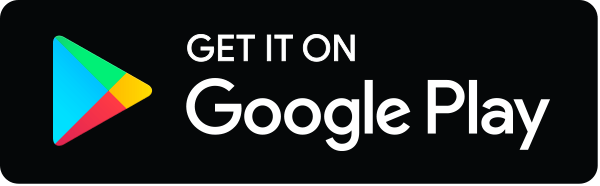
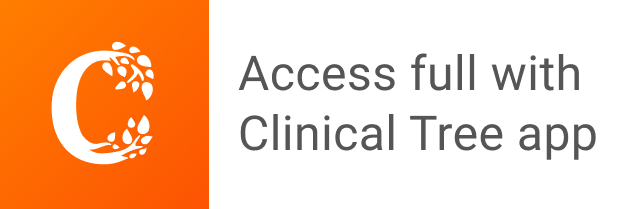