Chapter 6 Forelimb kinematics are described in terms of temporal (timing), linear (distance) and angular variables. In order to interpret data describing segment and joint angles, it is important to know where the angles were measured. For example, joint angles may be measured between the proximal and distal segments on the anatomical flexor aspect or as the angle by which the distal segment deviates from alignment with the proximal segment, or some combination of these methods (Fig. 6.1). Furthermore, the angle may be expressed in absolute terms or it may be normalized to the standing angle, the angle at ground contact or the average angle during the stride (Mullineaux et al., 2004). GRF data can be combined with kinematic data using a link segment model to calculate internal forces within the limb that cannot be measured directly (see Chapter 19 for details). Briefly, in a two-dimensional link segment model, each segment is represented as a solid bar and the location of its center of mass is known relative to the coordinates that define the segment. The input for the model comprises kinematic and force data that are synchronized in time and space, together with segment morphometric data (Fig. 6.2, Table 6.1). An inverse dynamic solution is used to compute net joint moments and net joint powers (Colborne et al., 1997a,b). The net joint moment represents the net torque acting around a joint, which is produced primarily by the soft tissues (muscle, tendon and ligament). Net joint power, calculated as the product of the joint moment and that joint’s angular velocity, measures the rate of mechanical energy generation and absorption across a joint. Discrete bursts of positive and negative work can be quantified as the areas under the positive and negative phases, respectively, of the power curve. Positive work is done when the net joint moment acts in the same direction as the angular velocity of the joint, indicating that the muscle shortens as it generates tension (concentric contraction). Negative work is done when the net joint moment acts in the opposite direction to the angular velocity of the joint, so the muscle lengthens as it generates tension (eccentric contraction) and acts to restrain joint movement in opposition to gravity or some other external force. Table 6.1 Forelimb segmental masses, densities, reference lines for division of segments (see Fig. 6.2 for key to marker locations), and position of segmental centers of mass in the sagittal plane* *Position of center of mass is expressed along the x-axis (longitudinal, positive distally from the more proximal marker), then along the y-axis (perpendicular to x-axis, positive cranially). Distances are expressed as percentage segment length between the two reference markers. Location along the x-axis is measured first from the proximal reference marker toward the distal reference marker, then shifted along the y-axis. Data are mean ± SD for 12 forelimbs of 6 Warmblood horses. Reprinted from Willemen, M.A., Savelberg, H.H.C.M., Barneveld, A., 1997, The improvement of the gait quality of sound trotting warmblood horses by normal shoeing and its effect on the load on the lower forelimb, Livestock Production Science, 52 (2), 145–153, with permission from Elsevier. Fig 6.2 Skin markers used to locate the centers of mass of the forelimb segments in Table 6.1, which are separated according to the incision lines shown in red. Reprinted from Buchner, H.H.F., Savelberg, H.H.C.M., Scharmhardt, H.C., Barneveld, A., 1997, Inertial properties of Dutch Warmblood horses, Journal of Biomechanics, 30 (6), 653–658, with permission from Elsevier. Locomotor muscles account for about 42% of the horse’s body mass (Gunn, 1978) with the large, powerful muscles concentrated in the proximal limb, while the distal forelimb makes use of long, elastic tendons to reduce the metabolic cost of locomotion. The bulk of the musculature is in the proximal limb, which reduces the moment of inertia of the limb as a whole. The functions of the musculotendinous system of the equine forelimb include connecting the forelimb to the trunk; supporting the body mass; stabilizing the joints in opposition to the force of gravity during the stance phase; generating forces that are used for propulsion, braking and turning; and flexing the joints to lift the hoof clear of the ground during the swing phase. At first glance, there appears to be considerable redundancy in muscular function but muscles with similar attachments may, in fact, have quite different functional responsibilities. Evaluation of the geometry and architecture of the musculotendinous units is helpful in understanding whether their function is to produce rapid movements or generate large forces to stabilize the joints. Muscle size is expressed in terms of its mass and volume, which are closely correlated. Muscle density, calculated as mass divided by volume, has been determined to be 1.075 g/cm3 over a range of muscles, with different muscles varying by only a small amount (Brown et al., 2003). Fiber length and pennation angle affect the range of motion through which the muscle contracts and its ability to generate force. Long fibers arranged in parallel with the long axis of the muscle belly have the greatest capacity to shorten the muscle. Thus parallel fibers impart the largest range of motion and the most rapid shortening velocities. These characteristics are associated with muscles that act as prime movers. When fibers insert into the tendon at an angle (pennation angle), the amount of force transmitted to the tendon is determined as force developed in the fiber multiplied by the cosine of the pennation angle. Thus, fibers with a pennation angle of 40° transmit only 77% of the force generated by the muscle fiber to the tendon. In the equine antebrachial muscles, a close inverse relationship between muscle fiber length and pennation angle has been demonstrated (Brown et al., 2003), and this relationship may be true for other anatomical areas. The force of contraction of a muscle can be estimated in a Hill-type model based on four parameters: fiber length, maximal fiber shortening velocity, pennation angle, and peak isometric muscle force (Zajac, 1989). In general, muscle size (volume and mass) and fiber length decrease in a proximal to distal direction within the equine limbs. Muscles in the proximal forelimb tend to be large and powerful with long fibers arranged parallel to the muscle belly to move the joints through a large range of motion. By comparison, muscles in the distal limb are smaller and less powerful with short, pennate fibers that are not capable of a large amount of shortening but are well suited to contract isometrically. When muscle length is controlled by an isometric contraction, the long tendons are loaded elastically as the limb accepts weight in early stance, then recoil to release the stored elastic energy at the end of stance. Large, cursorial animals use this musculotendinous arrangement to move at high speeds with a relatively economical metabolic cost (Alexander, 2002). The extrinsic muscles of the forelimb, which have an attachment to the bones of the limb and an attachment to the trunk, are responsible for suspending the trunk between the forelimbs and for moving the forelimbs relative to the trunk. In general, these are large muscles with long fibers arranged parallel with the long axis of the muscle belly (Table 6.2) that insert on the bones via short tendons or aponeurotic sheets (Payne et al., 2004). This internal architectural style favors the ability to contract rapidly through a wide range of motion at the expense of the ability to generate high forces. The exception is serratus ventralis thoracis, which has short, pennate fibers and is encased in a strong aponeurotic sheath. These features suggest that serratus ventralis thoracis bears primary responsibility for anti-gravitational support of the trunk, whereas the other extrinsic muscles move the forelimb relative to the trunk during the swing phase or advance the trunk over the grounded limb during the stance phase. Table 6.2 Architectural properties of the extrinsic muscles of the equine forelimb Reprinted from Payne, R.C., Veenman, P., Wilson, A.M., 2004, The role of the extrinsic thoracic limb muscles in equine locomotion, Journal of Anatomy, with permission from John Wiley and Sons. Serratus ventralis thoracis (Fig. 6.3, Table 6.2) is the largest extrinsic muscle of the forelimb both in mass and volume and has the shortest mean fiber length. It is a broad flat muscle covered medially and laterally by broad aponeurotic sheets. The shortness of the muscle fibers (49 mm) relative to the length of the aponeurosis (500 mm) offers limited ability for muscular contraction to compensate for stretching of the aponeurosis, which implies that this muscle would not be effective in moving or positioning the limb. The elasticity of the aponeurosis may contribute to the overall elastic properties of the limb by acting in series with the spring-like tendons in the more distal part of the limb and may support protraction of the scapula (Smythe et al., 1993). Pennation of the fibers and the consequent increase in cross-sectional area gives the muscle considerable passive stiffness and allows it to generate sufficiently high forces to withstand gravitational loading of the limbs during galloping. Serratus ventralis thoracis is the primary muscular component of the thoracic sling, which suspends the trunk between the forelimbs and controls the position of the thorax and withers relative to the scapulae when the forelimbs are weight-bearing. During standing, the trunk is supported passively by elongation of the muscle fibers and the series elastic elements. Much higher forces must be resisted during locomotion when gravitational and inertial loads on the limbs produce peak vertical forces of at least 8 kN per limb for a 500 kg horse (McGuigan & Wilson, 2003). Loading during locomotion is easily resisted by the maximal isometric force-generating capacity of serratus ventralis thoracis, which is estimated to exceed 17 kN. This muscle is more variable in its mass than the other extrinsic muscles, which may reflect adaptation in response to the amount and type of training. Fig 6.3 Extrinsic muscles of the forelimb. From Stubbs and Clayton (2008) with permission of Sport Horse Publications. Serratus ventralis cervicis (Fig. 6.3, Table 6.2) is somewhat smaller than the thoracic part of the muscle and differs in having relatively long fibers and a smaller cross-sectional area (Payne et al., 2004), that confer the ability to support the base of the neck or to retract the limb by rotating the proximal scapula cranially. In dogs, it has been suggested that the primary function of serratus ventralis cervicis is to stabilize the position of the fulcrum about which the forelimb rotates in a craniocaudal direction during active retraction of the forelimb, thus ensuring that the GRF vector passes close to the center of scapular rotation (Carrier et al., 2006). The pectoral muscles (Fig. 6.3, Table 6.2) are large with relatively long fibers that suggest a primary role in adducting the forelimb (Payne et al., 2004). The deep pectoral (pectoralis ascendens) is a large, powerful muscle with long fibers. It is active through most of the stance phase when it may assist in moving the trunk forward over the grounded limb. The superficial pectorals (pectoralis descendens and pectoralis transversus) are smaller muscles with medium length fibers (Payne et al., 2004). Subclavius (Table 6.2) has long fibers (519 mm) that allow generation of large forces to assist in adduction and retraction of the forelimb or stabilization of the scapula (Payne et al., 2004). Trapezius (Fig. 6.3) and rhomboideus are the smallest extrinsic muscles of the forelimb and have medium-length fibers (Table 6.2) (Payne et al., 2004). Both muscles insert on the dorsal scapula and have fiber orientations ranging from vertical in the middle of the muscle to almost longitudinal towards the extremities. The orientation of the vertical fibers suggests that their function is to hold the proximal scapula against the trunk (preventing winging of the scapula) by opposing the action of the pectoral muscles that adduct the distal scapula and humerus. The longitudinal fibers likely contribute to forelimb protraction, retraction and stabilization. Brachiocephalicus (Fig. 6.3) and omotransversarius are considered together since their fibers cannot be separated close to their origins in the shoulder region. They form a large, powerful muscle, with long fibers oriented parallel to the muscle belly (Payne et al., 2004). Fiber lengths in Table 6.2 represent a mean of the two muscles, though brachiocephalicus has longer fibers than omotransversarius. The fiber direction suggests a primary role in forelimb protraction. Latissimus dorsi (Fig. 6.3, Table 6.2) is a moderately large and powerful muscle with fairly long fibers (Payne et al., 2004). It is enveloped by an aponeurotic sheath that is part of the thoracolumbar fascia. The fiber orientation suggests a primary role in forelimb retraction. Compared with the primary limb protractors, brachiocephalicus and omotransversarius, latissimus dorsi develops similar amounts of force but less than half as much power. Electromyographic studies indicate that this muscle is active in late swing and at hoof contact (Preedy, 1998), when it may act to retract the limb in preparation for ground contact and stabilize the trunk during the impact phase. The intrinsic muscles of the forelimb are characterized by being smaller in volume than the extrinsic musculature with short, highly pennate fibers and long tendons relative to muscle length. Total mass of the intrinsic musculature is around 17 kg in each forelimb. Pennation of the muscle fibers results in a larger PCSA than for equal-sized muscles with parallel fibers. This confers the ability to resist elongation of the muscle (isometric contraction) as the limb is loaded, so elongation of the musculotendinous unit is due to stretching of the tendon that acts in series with the muscle. As the tendons stretch, they store elastic energy, which is released later in the stance phase when the limb is unloaded. This mechanism reduces muscular work and increases the economy of locomotion (Alexander, 2002). Supraspinatus, with a mass of 793–1546 g and fiber length of 5–12 cm, has limited force generating capacity and a small moment arm at the shoulder, which makes it more suitable for stabilization than dynamic movement of the joints (Watson & Wilson, 2007). Supraspinatus and infraspinatus are active during early and midstance in walk, trot and canter (Aoki et al., 1984; Robert et al., 1998), when the primary action of supraspinatus appears to be stabilization of the shoulder joint. The instability seen with paralysis of the supraspinous nerve (Sweeney) supports this presumptive function. The medial and lateral heads of the biarticular biceps brachii span the extensor aspect of the shoulder and the flexor aspect of the elbow. The lateral head, with a mass of 171–343 g, is composed of short (5–8 mm), pennate fibers (Watson & Wilson, 2007), a large percentage of which are slow-twitch and well suited to postural control. The medial head, which has longer fibers (15–40 mm) and fewer slow-twitch fibers, may be more important in locomotion (Hermanson & Hurley, 1990). Compared with supraspinatus, biceps brachii has a larger force generating capacity and a larger moment arm at the shoulder joint, which suggests that it may be a more effective extensor of the shoulder (Watson & Wilson, 2007). A strong internal tendon (mass, 122–260 g; fiber length, 9–17 cm) runs through the muscle belly of biceps brachii uniting the tendons of origin and insertion. This tendon plays an important role in elastic energy storage, having the potential to store 277–591 J. It is estimated to withstand forces of 3.2 × 104 − 5.4 × 104 N when stretched as the forelimb is retracted in late stance with the shoulder and elbow in extension (Watson & Wilson, 2007). Recoil of the stretched biceps tendon in the galloping horse has been described as a catapult mechanism that provides rapid acceleration of the distal forelimb (Wilson & Watson, 2003). In a catapult, a large force is applied to store energy, which is then released rapidly to accelerate a small mass. In the horse’s forelimb, the biceps tendon is stretched by forward movement of the trunk and the changing orientation of the ground reaction force vector relative to the shoulder and elbow joints during the stance phase. Stretching continues as long as the carpus is locked in extension. When the carpus buckles, the forearm is released allowing the biceps tendon to recoil. The effect is rapid extension of the shoulder, flexion of the elbow and forward acceleration of the distal limb. Tendons can recoil elastically much faster than muscles shorten, which is beneficial in situations where rapid movement is required. In a galloping horse, the biceps tendon has been estimated to release 243 J in 0.11 s, which would require the power output of 50 kg of non-elastic muscle (Wilson & Watson, 2003). Thus the biceps catapult is an effective and efficient mechanism for protracting the forelimb in galloping horses. A further benefit of using tendon elasticity rather than muscular force is that the tendon has low energy dissipation, returning an impressive 93% of the work done stretching it and with only 7% dissipation as heat. Part of the internal tendon of biceps brachii emerges from the muscle and continues distally as the lacertus fibrosus, a tendinous band that blends with the epimysium of extensor carpi radialis. Lacertus fibrosus has a much smaller mass than the internal tendon of biceps brachii and is capable of storing much less energy (10–28 J) (Watson & Wilson, 2007). These properties are consistent with its role in stabilizing the forelimb as part of the stay apparatus. Biceps brachii has tonic activity during standing (Tokuriki et al., 1989), which supports the suggestion that the lateral part of the muscle, with its high proportion of type I muscle fibers, acts in series with lacertus fibrosus and extensor carpi radialis to stabilize the shoulder as part of the passive stay apparatus (Hermanson, 1997). During locomotion at walk, trot and canter, biceps brachii is active in early and midstance (Tokuriki et al., 1989). This is in contrast to brachialis, which acts as an elbow flexor in early swing (Tokuriki et al., 1989) and contributes to the flexor moment at the elbow at this time in walk (Clayton et al., 2000a, b) and trot (Lanovaz et al., 1999). Triceps brachii is a biarticular muscle crossing the flexor aspect of the shoulder (long head) and extensor aspect of the elbow. It is by far the largest of the intrinsic forelimb muscles. As the name suggests, it has three heads. The biarticular long head (mass, 3200–6663 g; fiber length, 19–26 mm) and the monoarticular lateral head (mass, 514–1240 g; fiber length, 17–24 mm) comprise 81% and 15%, respectively, of the extensor muscle mass at the elbow (Ryan et al., 1992). They contain predominantly fast-twitch fibers suggesting they are important in locomotion. By contrast, the small medial head of triceps (mass, 85–271 g; fiber length, 9–17 cm) and the anconeus muscle each account for only 2% of elbow extensor muscle mass and both are composed almost entirely of slow-twitch fibers, suggesting their role is to support the elbow in extension during stance (Ryan et al., 1992). The actions of triceps are to extend the elbow, to retract and extend the distal forelimb and, perhaps, to extend the limb when it is being used to raise the forehand. It is active in late swing and early stance (Tokuriki et al., 1989; Preedy, 1998; Robert et al., 1998), with activity in the long head preceding activity in the lateral head. Since biceps brachii and the long head of triceps brachii are biarticular, their interaction affects motion and stability of both shoulder and elbow joints. The nature of this interaction differs between the inverted pendulum behavior of the limb during walking and the mass-spring behavior during trotting. In the walking horse, the shoulder acts primarily as an energy damper, with a large burst of energy absorption on its extensor (cranial) aspect in midstance (Clayton et al., 2000a), which is likely due to eccentric action of biceps brachii controlling extension of the shoulder. At the elbow there are bursts of energy generation on the extensor aspect in early stance, which is thought to be due to concentric action of triceps brachii, and on the flexor aspect in late stance, which coincides with electrical activity in biceps brachii (Tokuriki et al., 1989). During trotting, the elbow has well-defined bursts of energy absorption on the extensor side of the joint in early stance followed by energy generation on the extensor aspect in midstance (Clayton et al., 1998). Energy absorption and generation on the extensor (cranial) aspect of the shoulder joint are similar in magnitude to those at the elbow joint but occur slightly later in the stance phase. There is an additional burst of energy generation on the extensor aspect of the shoulder in late stance (Clayton et al., 1998) corresponding with activity in biceps brachii (Tokuriki et al., 1989). Thus biceps brachii appears to be responsible for a net extensor moment at the shoulder through most of stance, while triceps brachii is generating an extensor moment at the elbow. In the swing phase, forelimb protraction is driven by the elbow flexors, which generate a flexor moment in early swing, and retraction is driven by the elbow extensors, which generate an extensor moment in late swing (Lanovaz et al., 1999). The muscles of the forearm move and stabilize the carpal and digital joints. The architectural properties of these muscles have been described (Hermanson, 1997; Hagen et al., 2002; Brown et al., 2003; Zarucco et al., 2004) and are summarized in Table 6.3. The carpal flexors and extensors have longer muscle bellies with shorter tendons than the digital flexors and extensors. The carpal net joint moment acts on the palmar aspect through most of stance, suggesting that tension in the carpal flexors assists the passive tendinous structures in maintaining joint stability (Clayton et al. 1998, 2000a, b). Flexor carpi ulnaris and ulnaris lateralis have short, highly pennated fibers (pennation angle close to 30o), which results in a large PCSA (Brown et al., 2003). Both of these muscles insert on the accessory carpal bone, which increases their moment arm and facilitates their ability to stabilize the carpus during stance. These muscles show electromyographic activity in late swing and early stance (Jansen et al., 1992) indicating a possible role in stabilizing the carpus through the impact phase. (Note that ulnaris lateralis represents the extensor carpi ulnaris but, since its distal attachment is to the accessory carpal bone in horses, it acts as a carpal flexor rather than a carpal extensor.) Table 6.3 Architectural properties of the muscles of the equine antebrachium MFL, mean fiber length; PCSA, physiological cross sectional area; MPA, mean pennation angle. Reprinted from Brown, N.A.T., Kawcak, C.E., McIlwraith, W., Pandy, M.G., 2003, Architectural properties of distal forelimb muscles in horses, Equus caballus, Journal of Morphology, with permission from John Wiley and Sons. Flexor carpi radialis and extensor carpi radialis (Table 6.3) have long muscle fibers with small pennation angles (less than 20°), a small PCSA, and short tendons (Brown et al., 2003). These qualities suggest a role in initiating and controlling carpal flexion/extension during the swing phase. Extensor carpi radialis also shows evidence of a degree of compartmentalization; the proximal portion has a predominance of fast-twitch fibers that can contract powerfully, while the distal portion has more slow-twitch fibers (Hermanson, 1997). The fatigue resistant slow-twitch fibers arranged in series with the tendons of biceps brachii and lacertus fibrosus are part of the passive stay apparatus of the forelimb. Electromyographic activity in extensor carpi radialis is concentrated at the beginning of swing (Jansen et al., 1992) when the elbow and carpus are flexing. At this time, the net joint moment is on the cranial side of the joints (Lanovaz et al., 1999) indicating that the biarticular extensor carpi radialis is flexing the elbow to protract the limb and is controlling the inertially driven carpal flexion (Colborne et al., 1997a, b). Rupture of the extensor carpi radialis tendon allows the carpus to hyperflex during the swing phase at walk and may cause the horse to stumble or fall at trot because it cannot protract the forelimb rapidly enough. Later in the swing phase, the net joint moment moves to the caudal side of the carpus (Lanovaz et al., 1999), with flexor carpi radialis controlling carpal extension and retracting the distal limb in preparation for ground contact. The digital flexor and extensor muscles (Table 6.3) are characterized by having long tendons relative to their muscle length. However, there are marked differences in muscle architecture and tendon properties of the deep and superficial digital flexor muscles that are indicative of the different roles played by these musculotendinous units in locomotion. The deep digital flexor (DDF) has three distinct muscle bellies, humeral, ulnar and radial, each of which is innervated by a separate branch of the median nerve suggestive of neuromuscular compartmentalization (Zarucco et al., 2004). The DDF is also compartmentalized morphologically into regions with different lengths of fibers in the range 5–117 mm (Hagen et al., 2002; Brown et al., 2003; Zarucco et al., 2004). The humeral head, which comprises about 74% of total muscle volume, has the longest fibers of any muscle in the antebrachium, but also has some short and medium length fibers. Its tendon is long, but not as long as that of the ulnar head, which also has long fibers but relatively small mass and PCSA compared with the humeral head. In contrast, the fibers in the radial head are short and highly pennated (pennation angle close to 30°). The functions of the DDF are to flex the digital joints during the swing phase and to generate a propulsive force during the second half of stance. Since the DDF muscle has a relatively high percentage of fast-twitch fibers, it is susceptible to fatigue during exercise (Hermanson & Cobb, 1992; Butcher et al., 2007). The DDF tendon, which functions as a positional tendon, has a higher modulus of elasticity than the SDF tendon, which is used for elastic energy storage and release (Birch, 2007). At the walk, the deep head of DDF is active throughout stance with peaks of activity in early stance and late stance (Jansen et al., 1992). Horses with palmar foot pain may prolong the activation of DDF in early stance to move the center of pressure beneath the hoof in a dorsal direction, thereby relieving weight-bearing in the palmar part of the hoof (Wilson et al., 2001). The superficial digital flexor (SDF) muscle has a much smaller volume than the DDF muscle. However, the fibers are very short (2–10 mm) and highly pennate (pennation angle up to 60°) (Grandage, 1981; Dimery et al., 1986; Hermanson & Cobb, 1992; Biewener, 1998; Wilson et al., 2001; Brown et al., 2003; Zarucco et al., 2004), resulting in the largest cross-sectional area of the antebrachial muscles and endowing a large force-generating capacity (Hagen et al., 2002; Brown et al., 2003). As a consequence of the large pennation angle, the SDF is capable of minimal shortening and only about 71% of the total muscle force is transmitted to the tendon. The SDF muscle has a high percentage of slow-twitch muscle fibers (Butcher et al., 2007) that are resistant to fatigue. This fiber composition is well suited for its support role as part of the stay apparatus (Swanstrom et al., 2005), and for attenuating high-frequency forces associated with impact (Wilson et al., 2001). The fatigue-resistant, slow-twitch fibers of the SDF tendon act eccentrically or isometrically during stance with changes in length of the musculotendinous unit being due almost entirely to stretching of the elastic tendon (Butcher et al., 2007). The third interosseous muscle (suspensory ligament) acts as an energy-storing tendon and has an even lower modulus of elasticity than the SDF tendon (Birch, 2007). It supports the metacarpophalangeal (MCP) joint during the stance phase, which is critical to the function of the equine limb. During standing, the suspensory ligament is fully capable of supporting the horse’s weight passively (Dyce et al., 1996). It has been speculated that reduction in the muscular function of the equine interosseus began about 15 million years ago, when ancestral horses were increasing in size and moving to the grasslands where efficient overground locomotion was required (Camp & Smith, 1942). Today, muscle fibers comprise about 10% of the suspensory ligament. These muscle fibers are short (~0.88 mm) and highly pennate (pennation angles >45°), which increases the PCSA and contributes to the ability to generate forces but produces little work. The vast majority (95%) of the muscle fibers are type I, and presumably slow-twitch (Wilson et al., 2001; Soffler & Hermanson, 2006). Thus, the muscular content of the suspensory ligament is small but significant and the architecture of the fibers suggests that it contributes to forelimb stability and elastic energy storage during locomotion. The common digital extensor (CDE) (Table 6.3) and lateral digital extensor muscles have long fibers, small PCSA and long tendons (Brown et al., 2003). The CDE muscle is active in terminal swing at the walk, when it extends the digit in preparation for ground contact, (Jansen et al., 1992). The CDE tendon has a stiffer matrix than the SDF tendon, which may be due to the smaller fibril diameters (Birch, 2007). As horses bounce over the ground in the trot, canter and gallop, the forelimbs have been estimated to contribute one-third of the energy storage compared with two-thirds in the hind limbs (Biewener, 1998). During trotting, changes in potential and kinetic energy of the horse’s center of mass are in phase, which allows the distal limb to make substantial contributions to elastic energy storage (Biewener, 1998). In canter and gallop, the relationship between kinetic and potential energy varies during the stride (Minetti et al., 1999), which limits the ability to store elastic energy in the distal limb. At faster speeds, vertical excursions of the center of mass are reduced and the limb sweeps through a larger angle during its stance phase causing the horse to bounce off the ground more quickly (Farley et al., 1993). The concept of the forelimb acting as a spring implies that changes in joint angles as the limb accepts weight result in shortening of the bony column and stretching of the musculotendinous units. During galloping, the proximal limb from scapula to elbow shortens by about 12 mm, whereas the limb distal to the elbow shortens by around 127 mm. One of the most obvious articular changes is extension of the MCP joint, the magnitude of which can be predicted from vertical ground reaction force (McGuigan & Wilson, 2003). In general, the limb spring is stiffer in larger animals but, within an individual, stiffness of the limb spring is nearly independent of speed. In the equine distal limb, the suspensory ligament and SDF tendon are primarily responsible for storing and releasing elastic energy. They are subject to high tendon strains as the limb is loaded during stance, especially at the trot. In the canter, overall limb loading decreases with less elastic energy being stored in the SDF tendon, and the DDF tendon being more loaded (Butcher et al., 2007). With an increase in galloping speed, the DDF muscle and its associated tendon assume a greater role in support of the MCP joint, thus relieving some stress on the SDF tendon. The DDF muscle, however, has fast-twitch fibers that contract concentrically and are susceptible to fatigue. When the DDF muscle becomes fatigued, the SDF tendon is over-loaded and predisposed to strain injury (Butcher et al., 2007), which occurs frequently in equine athletes, especially in Thoroughbred racehorses (Peloso et al., 1994). Even though the elastic tendons are highly energy efficient and dissipate only 7% of the stored elastic energy as heat, temperatures around 45°C have been found in the SDF tendon of racehorses after galloping (Birch et al., 1997). Repeated loading of the musculotendinous unit during training and racing may lead to the accumulation of subclinical microdamage if the processes of repair and adaptation are unable to keep up with the rate of tissue damage (Hill et al., 2001). Hoof angle affects strain distribution between the tendoligamentous structures in the distal limb. Elevation of the heels reduces peak strain in the DDF tendon and increases peak strain in the SDF tendon and suspensory ligament, whereas raising the toe has the opposite effects (Lawson et al., 2007). The joints of the horse’s forelimb from the elbow distally are more or less constrained to move in a sagittal plane with relatively small amounts of abduction/adduction and internal/external rotation (Thompson et al., 1992; Degueurce et al., 1996). Studies of three-dimensional forelimb kinematics, which will be described at the end of this chapter, have confirmed that flexion/extension is the dominant rotation at the carpal, metacarpophalangeal, proximal interphalangeal (PIP) and distal interphalangeal (DIP) joints (Chateau et al., 2004, 2006; Clayton et al., 2004, 2007a, b; Hobbs et al., 2006). Inverse dynamic analysis has been used to calculate net moments of force, net joint powers and net joint energies for the joints of the equine forelimb at walk and trot (Hjertén & Drevemo, 1993; Clayton et al., 1998, 2000a, b; Colborne et al., 1998; Lanovaz et al., 1999; Hodson et al., 2000; Khumsap et al., 2002, Dutto et al., 2006). When using skin markers to represent two-dimensional motion of the limb segments, a minimum of two markers per segment is required. Typical marker configurations involve either placing a marker over the center of rotation of each joint or aligning two markers along the long axis of each segment (Fig. 6.4). Intra-limb coordination patterns can be visualized using stick figures or joint angle–time graphs (Fredricson & Drevemo, 1972; Fleiss et al., 1984; Martinez-del Campo et al., 1991; Holmström et al., 1994; Back et al., 1994; Degueurce et al., 1997; Nicodemus & Holt, 2006; Martuzzi et al., 2007; Nicodemus & Booker, 2007). Skin displacement relative to the underlying bones is always a concern when kinematic studies are based on skin-fixed markers. In the equine forelimb, artifacts due to skin displacement may be small enough to be negligible on the antebrachial and metacarpal segments, but are large enough to alter sagittal plane kinematics significantly on the scapular, brachial, and pastern segments. Since skin displacement has a cyclic pattern, it has been possible to develop mathematical correction algorithms for many of the anatomical locations that are commonly used for marker placement (van Weeren et al., 1990a, b; Sha et al., 2004). The trot is the most important gait for evaluation of the quality of a horse’s movement and for detection of lameness. It is a two-beat gait with the limbs coordinated by diagonal pairs. The diagonal support phases are usually separated by aerial or suspension phases in which all feet are off the ground (Alexander & Jayes, 1978). Back et al. (1995a) used standardized procedures to describe sagittal plane kinematics of the trot in a large group of Warmblood horses. The joint angle–time diagrams were analyzed simultaneously with corresponding stick figures and marker diagrams to create a complete picture of equine forelimb motion at the trot that could be related to limb function (Back et al., 1995a). Variability between horses was evaluated, and the effect of correcting for skin displacement on joint angle–time diagrams was quantified and illustrated graphically (Fig. 6.5) (van Weeren et al., 1990a; Back et al., 1995a).
Forelimb function
Terminology
Musculotendinous architecture
Extrinsic muscles of the forelimb
Intrinsic muscles of the forelimb
Sagittal plane analysis of forelimb kinematics and kinetics
The trot
Forelimb function
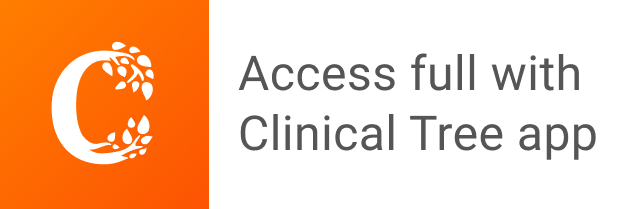