7 Fat and fatty acids
Structural chemistry and nomenclature
Similar to other classes of nutrients, fats are chemically and structurally diverse. The structure of fat has direct bearing on its physical properties, as well as its biological behavior and activity. The naturally-occurring fats in forages and cereal grains exist as a mixture of simple lipids (di- and triacylglycerol, nonesterified fatty acids, waxes and sterols) and complex lipids (glycolipids, and phospholipids) (Hargin & Morrison 1980, Harwood 1996, Zhou et al 1999). By comparison, fats and oils added to equine diets consist mostly of triacylglycerols, which are also commonly referred to as triglycerides. Triacylglycerols consist of three fatty acids esterified to a 3-carbon glycerol molecule (Fig. 7.1). Each fatty acid is a hydrocarbon chain, which can vary in length from 2 to 28 carbons. However, the esterified fatty acids present in most feedstuffs are usually 12 to 22 carbons in length. Nonesterified fatty acids (NEFA) unbound from the glycerol backbone typically make up a very small portion of the fat. In fact, the presence of such “free” fatty acids in a feed or oil is used as an indicator of fat rancidity (oxidation).
Besides length, fatty acids can also differ in their degree of saturation or unsaturation (Figure 7.1). Fatty acids that contain no double bonds between carbon atoms are referred to as saturated fatty acids. Fatty acids with one or more double bonds are referred to as mono- or polyunsaturated fatty acids, respectively. Saturated fatty acids have a high melting point. Fat sources rich in saturated fatty acids, such as animal lard or tallow are solid at room temperature. In contrast, unsaturated fatty acids have a lower melting point, making the fat liquid (i.e., oil) or near liquid at room temperature. Most plant-based fats have relatively high unsaturated fatty acid content; notable exceptions are coconut oil and palm oil. In practice, the term “fat” is commonly used when referring to either solid fats or liquid oils. The usage of “fat” in this chapter will collectively represent the fatty acids, triacylglyercol and other complex lipid components in feeds and oils.
Each fatty acid has a carboxylic acid group at one end of the carbon chain and a methyl group at the other terminus. The carboxyl carbon is referred to as the delta (Δ) carbon, whereas the methyl carbon is the omega (ω or n) carbon (Fig. 7.1). Both of these landmarks have been used to reference the position of the double bond(s) in a fatty acid (i.e., the numbering of carbons in the chain can begin at either the Δ or ω carbon). The reference to the omega carbon is often favored when describing essential fatty acids. However, the international nomenclature system preferred by lipid biochemists references the position of double bonds from the delta terminus.
The length of a fatty acid, its degree of unsaturation, and the position of the double bonds within the fatty acid all dictate its biological function. As mentioned, there are numerous methods of nomenclature to identify each fatty acid based on these characteristics. In this chapter, the trivial name of the fatty acid (e.g., linoleic acid) and the shorthand numerical symbols are provided. The latter will consist of two numbers separated by a colon. The number to the left of the colon is the number of carbons in the fatty acid chain and the number to the right denotes the number of double bonds. Where appropriate, the specific omega family (e.g., n-3, n-6) that the fatty acid belongs to is also noted. For example, the shorthand nomenclature for linoleic acid is 18:2n-6, indicating it is an 18-carbon fatty acid with two double bonds and is a member of the omega-6 family (Fig. 7.1). Although the nomenclature system presented herein is susceptible to some ambiguity, it remains the most widely used for identifying specific fatty acids in the scientific and popular literature. The reader is referred elsewhere for a more detailed tutorial on fatty acid nomenclature, including the more formal naming system recognized by the International Union of Pure and Applied Chemists and the International Union of Biochemistry (IUPAC-IUB 1976, Fahy et al 2005).
Key Points
• Dietary fats are chemically and structurally diverse, and may include triacylglycerol, phospholipids, glycolipids and other lipids.
• Plant sources of fat most often consumed by horses are generally rich in unsaturated fatty acids.
• The omega nomenclature system identifies the placement of double bonds from the methyl end of the fatty acid chain.
Fat digestion and absorption
Digestive physiology
Enzymatic digestion of fat begins in the stomach. In horses, the zymogen (chief) cells of the fundic mucosa are known to produce large amounts of gastric lipase that exhibits peak activity at pH 4 (Moreau et al 1988). While it is presumed that gastric lipase initiates hydrolysis of triacylglycerols, the extent of fat lipolysis taking place in the equine stomach is unknown. In other species, the fatty acids released in the stomach are thought to be involved in priming the release of gastrointestinal hormones, which contribute to the emulsification of fat in the duodenum. Cholecystokinin is secreted from the duodenal mucosa in response to partially digested fats and proteins, and triggers the release of bile and pancreatic enzyme secretion. Additionally, the presence of gastric acid in the upper small intestine triggers the release of secretin, which in turn stimulates secretion of bicarbonate from the pancreas.
The products of digestion present in the lumen of the duodenum are one of many factors thought to regulate the rate of gastric emptying. For many species, it is generally believed that fat causes a greater inhibition of gastric emptying than carbohydrate (Meyer et al 1986); however, conflicting results have been reported for equids. Wyse et al (2001) fed ponies a small concentrate meal (0.4–0.6 g/kg BW) of oats and bran to which was added 0, 35 or 70 ml of soybean oil, resulting in meals containing approximately 0, 24 or 38% fat, respectively. Both fat-added meals resulted in a significant delay in gastric emptying compared to a meal without oil as measured by the 13C-octanoic acid breath test. Using a similar technique, Geor et al (2001) found that the addition of corn oil (10%) to a sweet feed meal (2 g/kg BW) slowed gastric emptying in horses. Further, this effect was not influenced by the diet the horse was habituated to (with or without added fat), nor by a 4- or 8-week period of adaptation to a fat supplemented diet. By comparison, consumption of a high fat diet induces adaptation in humans, ultimately reversing the slowing effect of a fatty meal and enhancing gastric emptying (French et al 1995). Unfortunately, the specific role that fat plays in gastric emptying in horses is somewhat confounded in the studies of Wyse et al (2001) and Geor et al (2001), as control and fat-added test diets were not isocaloric. In other species, meal volume, caloric density, viscosity, and osmolality have also been shown to influence gastric motor function (Meyer et al 1986). A possible effect of viscosity was noted by Wyse et al (2001), where a delay in gastric emptying similar to that caused by the addition of soybean oil was observed when an indigestible oil (Olestra®) was added to the test meal. In contrast to these results, a series of studies performed by Lorenzo-Figueras et al (2005) demonstrated that small (0.5 g/kg BW) isocaloric meals high in carbohydrate or containing 8 or 12.3% fat had similar effects on gastric emptying (measured with the 13C-octanoic acid breath test) and proximal gastric tone (measured with an intragastric barostat) in horses. Additional research is needed to clarify the effects of fat on gastric emptying in horses, as the mechanism may differ from other monogastrics that routinely consume greater amounts of dietary fat.
The proposed process of fat digestion and absorption in the small intestine is depicted in Fig. 7.2. As the acidic fat emulsion leaves the stomach and enters the duodenum, it is neutralized and modified by mixing with bile and pancreatic juice. The horse lacks a gall bladder to store bile, and instead bile is continuously secreted by the liver directly into the duodenum. Pancreatic fluid supplies bicarbonate to neutralize the acidic chyme as well as enzymes that cleave fatty acids from triacylglycerol, phospholipids and cholesterol esters. On a relative basis, the equine pancreas produces much more lipase than any other digestive enzyme (e.g., amylase, trypsin) and the activity of pancreatic lipase has been shown to be similar between adult horses, pigs and rats (Lorenzo-Figueras et al 2007). Pancreatic lipase catalyzes the hydrolysis of fatty acids from the outer positions (sn-1 and sn-3) of triacylglycerol, yielding two NEFA and one monoacylglycerol. The activity and efficiency of pancreatic lipase are enhanced by bile salts and pancreatic colipase. Bile salts accumulate on the surface of lipid droplets, increasing the surface area of the oil–water interface and permitting water-soluble lipase to be effective. Colipase ensures adsorption of lipase to the emulsified substrate by acting as an anchor.
The products resulting from lipid hydrolysis in the small intestine assemble into mixed micelles. Micelles contain a monolayer of bile acids, monoacylglycerol and lysophospholipids, which surround a core of NEFA, cholesterol and fat-soluble vitamins. Micelles deliver the lipids to the brush border of the small intestine and, because of their small size, are thought to pass readily into the unstirred water layer at the surface of the microvillus membrane where they release NEFA and monoacylglycerol. A protein-independent diffusion model and protein-dependent mechanisms have been proposed for the uptake and transport of fatty acids across the apical membrane of the enterocyte, although the relative contribution of each mechanism to fatty acid uptake is still unknown (Mansbach & Gorelick 2007). In humans, FAT/CD36 and FABPpm have been identified as fatty acid transport/binding proteins that are important for fatty acid uptake at the intestinal brush border (Mansback & Gorelick 2007, Iqbal & Hussain 2009).
Once inside the enterocyte, fatty acids are transported via cytosolic fatty acid transport/binding proteins to the endoplasmic reticulum where they are re-esterified to form triacylglycerol and phospholipids. The nucleotide sequence of one of these, intestinal fatty acid binding protein (I-FABP), has been characterized in the horse and was shown to share 87% identity with human I-FABP (Nieto et al 2005). In healthy horses, the I-FABP gene was found to be highly expressed in small intestinal mucosa with low expression observed in the cecum and colon and virtually no expression in stomach mucosa (Nieto et al 2005).
For export into circulation, lipid products are repackaged within the enterocyte, creating a micellar particle with an outer monolayer of phospholipids, proteins and unesterified cholesterol, and a core of triacylglycerol, cholesteryl esters and fat-soluble vitamins. In most mammals, this enterocyte-derived lipoprotein is known as a chylomicron. However, it is unclear whether absorbed triacylglycerol is initially transported via chylomicrons in horses. A chylomicron-like lipoprotein has been identified in the non-fasting plasma of suckling foals, but not in mature horses or ponies maintained on forage and grain diets (see review by Watson et al 1993). Suckling foals were likely consuming more fat from mare milk compared to the mature horses evaluated. However, the fat content of the diet may not be the only factor influencing chylomicron formation as attempts to isolate this lipoprotein fraction from adult ponies following an acute oral fat load were unsuccessful (Watson et al 1993). In contrast, some investigators have reported very small concentrations of chylomicrons in the plasma of horses fed diets with or without added fat (e.g., Marchello et al 2000, van Dijk & Wensing 1989). However, the physical and chemical composition of the lipoprotein fractions were not described sufficiently in these studies to verify if these lipid fractions were in fact chylomicrons and not very low-density lipoproteins (VLDL). Although smaller and less dense, VLDLs are similar to chylomicrons in that they are triacylglycerol-rich. The lack of a chylomicron fraction in horses may reflect a mechanistic adaptation to relatively low fat, forage-based diets. In ruminants, dietary triacylglycerols are transported as modified VLDL when fed diets containing hay, silage and cereal grains, but chylomicrons are synthesized when large amounts of polyunsaturated fatty acids are infused into the small intestine (Byers & Schelling 1988). Even when present, ruminant chylomicrons are typically much smaller, lower in triacylglycerol, higher in phospholipids and less prevalent than described for humans (Byers & Schelling 1988). Differentiation of intestinally-derived chylomicrons (or modified VLDL) from liver-derived VLDL may prove difficult in the horse because the lipoprotein apo-B48, secreted exclusively by the intestine in most mammals, is also secreted by the liver in horses (Greeve et al 1993). Although horses are capable of absorbing dietary fat (see the “Fat Digestibility” section), more study is needed to elucidate the process of triacylglycerol transport from the small intestine and how these mechanism(s) may be modified by diet.
The absorption of dietary fat takes place primarily in the distal duodenum and jejunum. Triacylglycerol-rich lipoproteins (e.g., chylomicrons, VLDL) transport absorbed fats and deliver them to the liver, adipose and other tissues. In man, the half-life of a triacylglycerol carried by a chylomicron released from an enterocyte is about 5 minutes, whereas the half-life of a whole chylomicron particle is approximately 13 to 14 minutes (Gurr et al 2002).
Similar to other nutrients, fats that are not digested and absorbed in the small intestine will pass into the hindgut. Based on the difference in ether extract between ileal contents and feces, Swinney et al (1995) reported that little to no fat disappearance occurred in the hindgut of miniature horses fed diets consisting of 35% forage and 65% concentrate that contained 5 to 25% added fat. This agrees with reports in ruminants, where loss of longer chain fatty acids from the rumen either by absorption across the ruminal epithelium or by catabolism to volatile fatty acids or carbon dioxide is minimal (Jenkins 1993). However, dietary fats that escape digestion in the equine small intestine have the potential to interfere with microbial fermentation in the hindgut. The amount of fat entering the hindgut has been shown to increase with increasing intake of soybean oil. Consumption of a concentrate diet with 0, 3.9 and 11% soybean oil resulted in 11, 20 and 99 g fat/kg DM of chyme entering the cecum, respectively (Coenen 1986). When the 11% fat-added concentrate was administered via nasogastric tube transit through the stomach and small intestine was accelerated, resulting in a larger quantity of fat entering the cecum (146 g/kg DM) and evident alterations to the microbiota (Coenen 1986). In ruminants, where the subject has been extensively studied, the negative impact of dietary fat on fiber digestion is believed to result from either the coating of feed particles or a direct toxic effect on rumen microorganisms (Hess et al 2008). The type of dietary fat is also relevant, whereby unsaturated fatty acids have been shown to have greater antimicrobial effects and promote greater inhibition of ruminal fermentation when compared to saturated fatty acids (Hess et al 2008). Presumably the same events would occur in the hindgut of horses, but further investigation on the effects of dietary fat on microbial fermentation using different fat sources and hay to grain ratios is warranted.
Fat digestibility
The digestibility of dietary fat is primarily affected by level of intake and type of lipid. The low levels of naturally-occurring fat in forages and cereal grains appears to be the most difficult for the horse to extract, with apparent digestibility of fat ranging from 5–57% in forages (Fonnesbeck et al 1967, Sturgeon et al 2000) and 55–76% in grains (Hintz & Schryver 1989). In contrast, diets supplemented with various sources of animal fat or vegetable oil have an apparent digestibility between 64% and 96% (Kane et al 1979, McCann et al 1987, Rich et al 1981, Swinney et al 1995, Bush et al 2001). After compiling data from five studies evaluating five basal diets (hay-grain mix, 23–37 g fat/kg DM) and 18 diets with added fat (76–233 g fat/kg DM), Kronfeld et al (2004) reported mean apparent digestibilities of 55% for forages, 81% for mixed diets including added fat, and 95% for added fats. Further modeling of this data indicated that the true digestibility of added fats was nearly 100% (Kronfeld et al 2004).
The reason for the differences in digestibility of naturally-occurring vs. supplemental fat sources is likely multifactorial. Animal fats and vegetable oils supplemented to equine diets are mostly in the form of freely available triacylglycerols. By comparison, fats in forages and grains are surrounded by plant cell wall components which may delay or prevent presentation to lipase in the small intestine. Modeling of data from several feeding trials led Kronfeld et al (2004) to suggest that enzymatic hydrolysis of fat might be slower as a consequence of the low fat intakes associated with forage and grains. In addition, although routinely used, ether extract methodology may not adequately characterize the types of lipid found in forages and cereal grains. Two-thirds of the lipid content in forage (Harwood 1996) and up to one-third in cereal grains (Hargin & Morrison 1980, Zhou et al 1999) is in the form of glycolipids and phospholipids that are incompletely extracted in ether. Similarly, forages contain waxes and other pigments that are extractable in ether but poorly digestible. Thus, if digestibility of fat in forages and cereal grains is based on ether extraction, the availability of naturally-occurring fat in forages and cereal grains might be underestimated as a significant portion of the lipid is not represented in the extract. The relatively small amount of fat, coupled with incomplete characterization of the lipid fractions digested, might also explain the wide variation in fat digestibility commonly observed with forages (see Fonnesbeck et al 1967).
Despite the fact that the digestive system of equids has evolved to process low-fat, high-fiber feeds, they appear quite capable of assimilating relatively large amounts of dietary fat (see reviews by Potter et al 1992, Kronfeld et al 2004, NRC 2007). Using data from a wide range of fat intakes (23–233 g/kg DM) Kronfeld et al (2004), demonstrated a linear relationship between fat intake (g/day) and fat absorbed (g/day), with an estimated daily endogenous loss of 0.22 g fat/kg BW. Additional modeling of these compiled data demonstrated that fat digestibility is maximized between 100 and 150 g/kg DM and sustained to at least 230 g/kg DM in horses adapted to fat-supplemented rations (Kronfeld et al 2004).
Few associative effects of dietary fat on the digestibility of other nutrients have been reported. In the studies compiled by Kronfeld et al (2004), the feeding of diets with up to 230 g fat/kg DM had no negative effects on the digestion of dry matter, crude protein, or fiber. The supplemental fat sources and the percent fat added to the total diet in Kronfeld’s report included corn oil (5–20%), peanut oil (7.5–15%), tallow (7.5–15%), an animal–vegetable fat blend (7.5–15%), a blend of equal parts soy-lecithin and corn oil (10%), and a blend of equal parts soy-lecithin and soybean oil (10%). A similar lack of negative associative effects on nutrient digestibility have been reported by others with the addition of 5–15% corn oil (Kane et al 1979, Bush et al 2001, Lindberg & Karlsson 2001) and 8% linseed oil (Delobel et al 2008) to the concentrate portion of the diet. In contrast, a negative associative effect of soybean oil on crude fiber, neutral detergent fiber (NDF) and acid detergent fiber (ADF) digestion has been reported by Jansen et al (2000, 2001, 2002). In these studies soybean oil was added to the concentrate at a rate of 15–37%, resulting in 50–148 g fat/kg DM in the total diet. Other researchers have also found a negative effect on fiber digestion with fairly high levels of soybean oil inclusion in the concentrate portion of the diet (19–21%), but not lower levels (5–13%) (Godoi et al 2009, Morgado et al 2009). While reduced fiber intake associated with the feeding of high levels of soybean oil can possibly explain the reductions in fiber digestion observed by some (Godoi et al 2009, Morgado et al 2009), it does not explain the findings of Jansen et al (2000, 2001, 2002) who compared diets similar in energy and fiber by substituting corn starch and/or glucose for soybean oil. The mechanism by which soybean oil, but not other oils, might suppress fiber digestion is unknown. Higher fluxes of bile acids or fatty acids into the large intestine are not likely to be involved (Jansen et al 2007). A recent meta-analysis of 22 studies found that diets with added fat (15.5 to 217.5 g/kg DM) had no effect on digestion of crude protein or NDF but did result in a significant decrease in ADF digestibility (Sales & Homolka 2011). It is worth noting that this meta-analysis included the soybean oil supplementation studies of Jansen and coworkers. As ADF generally represents the least digestible fiber for the horse, the importance of this finding may have little practical relevance. To avoid potential negative effects on fiber digestion, the NRC (2007) recommended an upper limit of 0.7 g fat/kg BW (approximately 35 g/kg DM) when fat is supplemented in the form of soybean oil.
Only a small number of studies have evaluated the effect of fat supplementation on mineral absorption. In mature horses and ponies, absorption of calcium, phosphorus and magnesium appear to be largely unaffected by fat intakes of 80 to 98 g/kg DM (Davison et al 1991, McCann et al 1987, Meyer et al 1997). Although calcium absorption was not directly measured, growing horses fed a fat and fiber-based supplement (containing 11% corn oil) had lower radiographic bone density during the fall and winter months, but not the summer and spring months, compared to those fed an isocaloric supplement high in starch and sugar (Hoffman et al 1999). However, a follow-up study found no difference in radiographic bone density between weanlings provided fat and fiber when compared to starch and sugar-based feeds (Hoffman et al 2001). Given the potential for mineral availability to be decreased through the formation of fat-mineral soaps in the small intestine, additional study is needed to clarify the impact that high-fat diets have on the absorption of calcium and other minerals in growing horses.
The absorption of fat-soluble vitamins requires the presence of triacylglycerol and other fats in the small intestine. The impact of dietary fat on vitamin absorption has not been directly assessed in the horse. Kronfeld et al (2004) speculated that the lowered fat digestibility of traditional hay-grain diets may increase the risk of suboptimal vitamin A and E status. As evidence of this, ponies fed diets containing very low amounts of fat (0.05%) for 3 months were reported to have low plasma and tissue vitamin E concentrations, suggesting there may have been inadequate absorption of vitamin E (Sallmann et al 1991). The impact of dietary fat on vitamin absorption deserves further study, particularly as it may alter fat-soluble vitamin requirements in relation to dietary fat content.
Key Points
• The mechanics of fat digestion and absorption have received limited study in the horse.
• Dietary fat is principally digested and absorbed in the small intestine.
• Digestibility of fat from oils and other high fat feedstuffs is relatively high (85–100%).
• Inclusion of fat at up to at least 100 g/kg DM appears to have minimal impact on digestion of dry matter, crude protein, NDF or minerals.
Dietary sources of fat
The fat content of equine diets can be augmented by a variety of fat-rich ingredients. The crude fat and fatty acid composition of feedstuffs commonly included in equine diets is provided in Table 7-1. Horses have been reported to accept a variety of plant oils added to their diet (depending on the processing method used for extraction), including canola, coconut, corn, cottonseed, flax (linseed), olive, palm, peanut, rice bran, safflower and soybean oils (Bush et al 2001, Delobel et al 2008, Duvaux-Ponter 2004, Frank et al 2004, Gatta et al 2005, Hallebeek & Beynen 2002, Holland et al 1998, Kronfeld et al 2004, Lindberg & Karlsson 2001, Meyer et al 1997).
Table 7-1 Average Crude Fat and Fatty Acid Composition (g/kg DM) of Feedstuffs Commonly Fed to Horsesa,b
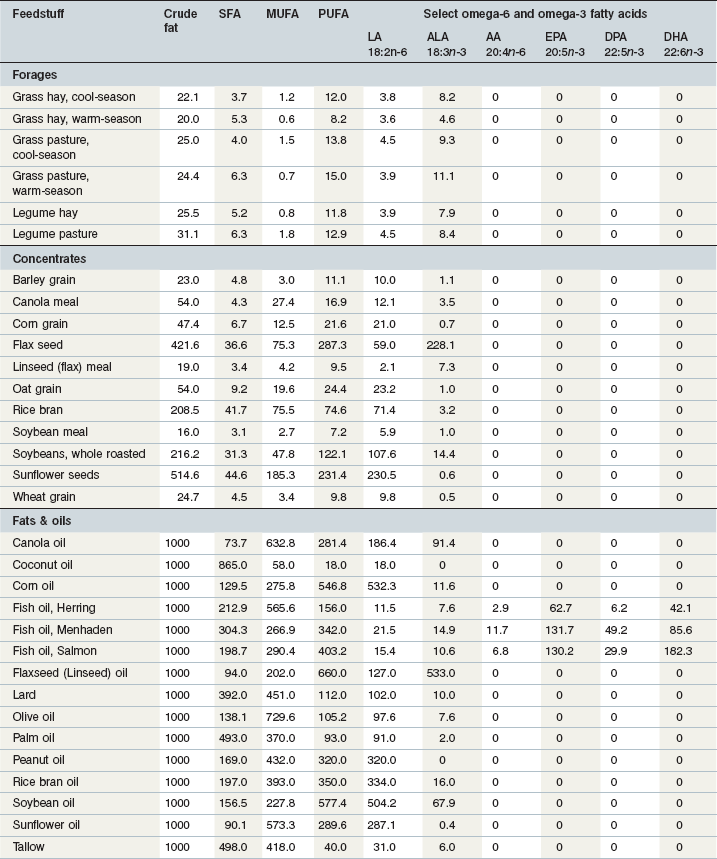
a SFA = saturated fatty acids; MUFA = monounsaturated fatty acids; PUFA = polyunsaturated fatty acids; LA = linoleic acid; ALA = α-linolenic acid; AA = arachidonic acid; EPA = eicosapentaenoic acid; DPA = docosapentaenoic acid; DHA = docosahexaenoic acid.
b Data from: Palmquist 1988, Boufaïed et al 2003, Warren & Kivipelto 2007a, b, USDA-ARS 2010.
Animal tallows and tallow-vegetable oil blends have also been used in horse rations (Holland et al 1998, McCann et al 1987, Rich et al 1981), although this practice is not as common today as it was 30 years ago and has been discouraged or prohibited in some countries based on perceived risk for transmission of bovine spongiform encephalopathy. In addition, palatability tests have generally indicated that horses have a preference for vegetable oils over animal fats (Bowman et al 1979, Holland et al 1998). More recently, interest in omega-3 fatty acids has led to the inclusion of marine fat sources in equine diets, including a variety of fish oils, seal blubber and algae to augment the omega-3 fatty acid content of the diet (Khol-Parisini et al 2007, King et al 2008, Vineyard et al 2010).
Key Points
• Forages and cereal grains that make up the bulk of most equine rations are generally low in fat (< 4% DM).
• Feedstuffs high in fat (20–100% DM) that can be added to equine rations include vegetable oils, marine oils, stabilized rice bran, flaxseed, and heat-treated soybeans among others.
• Horses have shown a preference for corn oil over other oils and vegetable oils over animal tallows and lards.
• Commercially available fat-added concentrates typically contain 5–14% crude fat.
Essential fatty acids
An EFA deficiency has not been described for the horse, even in those consuming diets almost devoid of fat. Sallmann et al (1991) observed no clinical abnormalities in ponies fed very low fat diets containing 0.03% and 0.14% linoleic acid for 7 months. The absence of signs of deficiency in these ponies may have resulted from mobilization of body fat stores that could have met EFA needs during the prolonged period of low intake. In other species EFA deficiency is characterized by dry or scaly skin, dry coat, hair loss and decreased reproductive efficiency. The NRC (2007) has recommended a LA intake of 0.5% DM for horses although justification for this recommendation was not described. For a 500 kg horse with a DM intake of 2% BW, the NRC recommendation would amount to a daily intake of 50 g of LA. Across a variety of other mammalian species, a minimum of 1% of total dietary energy intake as LA has been given as a general recommendation to prevent EFA deficiency (Gurr et al 2002). Extrapolating this to a 500-kg horse consuming 20 Mcal DE/day, and assuming a conservative estimate of 50% availability, the LA requirement is 45 g/day. Thus, the NRC (2007) recommendation for LA in horses appears to approximate the minimum intake guideline for other mammalian species. This requirement is likely to be met in horses consuming adequate quantities of good quality forage and is easily met by diets supplemented with fat, as most high-fat feedstuffs and oils are rich in LA (Table 7-1). Currently there are no guidelines for minimum daily ALA intake, although a horse consuming adequate amounts of fresh forage and/or good quality hay will likely receive ample amounts of ALA in the diet (Table 7-1). Supplementation with both LA and ALA should be considered in horses receiving poor quality or limited amounts of forage for prolonged periods of time. Because an ALA requirement has not been established for horses or other herbivores, provision of ALA in amounts resulting in a 5 : 1 to 10 : 1 ratio of LA : ALA might be considered adequate, as this has been recommended for other species (NRC 2005).
Key Points
• Linoleic acid (omega-6) and α-linolenic acid (omega-3) cannot be made by the horse and must be supplied by the diet.
• Essential fatty acid requirements have not been established for horses.
• Most equine diets will likely meet essential fatty acid needs unless poor quality rations are consumed for prolonged periods.
Omega-6 and omega-3 fatty acids
Both omega-6 and omega-3 fatty acids contribute to normal biological responses; however, the relative availability of omega-6 versus omega-3 fatty acids can influence the overall response. Linoleic acid (LA; 18:2n-6) is the “parent” omega-6 fatty acid and can be elongated and desaturated in the body to form the long-chain polyunsaturated fatty acids dihomo-γ-linolenic acid (DGLA; 20:3n-6) and arachidonic acid (AA; 20:4n-6) (Fig. 7.3). In the omega-3 family, α-linolenic acid (ALA; 18:3n-3) competes for the same elongase and desaturase enzymes to form eicosapentaenoic (EPA) and docosahexaenoic (DHA) acids. The initial step in this process catalyzed by Δ6-desaturase is thought to be rate-limiting, and studies in other species have indicated that there is a 1.5- to 3.0-fold higher Δ6-desaturase conversion rate for ALA compared with LA (Hussein et al 2005, Sprecher 2000, Sprecher et al 1995). The relative activities of Δ6- and Δ5-desaturase in horses have not been established. Nonetheless, horses appear to have the ability to synthesize the longer-chain polyunsaturated fatty acids from LA and ALA, as evidenced by the presence of AA, EPA and DHA in cell membranes despite not having consumed these fatty acids in the diet (Warren & Kivipelto 2008, Warren et al 2010).
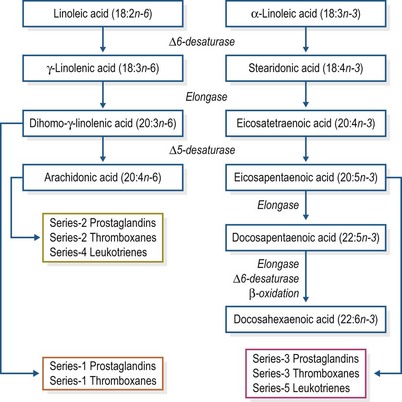
Figure 7.3 Elongation and desaturation of essential fatty acids and subsequent eicosanoid production.
In addition to competing for available enzymes, omega-3 and omega-6 fatty acids also compete for incorporation into cell membrane phospholipids. When greater quantities of EPA are present, it will be incorporated into cell membranes partially at the expense of AA (Calder 2006). The resulting changes in membrane fluidity and integrity, as well as cell receptor signaling and protein synthesis, can alter the biological response to trauma and infection. Furthermore, potent biological mediators known as eicosanoids are synthesized from the oxidation of EPA, DGLA and AA located in cell membranes. The eicosanoids, which include prostaglandins, leukotrienes and thromboxanes, mediate several events including inflammation, blood flow and pressure and blood clotting. DGLA and AA are precursors of the 1- and 2- prostaglandin and thromboxane series, whereas EPA serves as the precursor for the 3-series prostaglandins and thromboxanes and the 5-series leukotrienes (Fig. 7.3). In simplistic terms, eicosanoids derived from omega-6 fatty acids stimulate stronger pro-inflammatory responses, whereas those stemming from omega-3 fatty acids produce weaker inflammatory reactions. However, it is really the balance of the different eicosanoids produced that generates the final biological response. Because most cell membranes contain a greater concentration of AA than other 20-carbon fatty acids, AA is usually the principal precursor for eicosanoid synthesis (Calder & Grimble 2002).
Supplementation of omega-3 fatty acids
As an herbivore, the horse is adapted to a diet naturally high proportionally in omega-3 fatty acids. Forages, although low in total fat (2–4%), contain a significant portion of that fat (39–56%) as ALA (Boufaïed et al 2003, Warren & Kivipelto 2007a, b). In both fresh forage and hay, the proportion of ALA usually exceeds that of LA (Tables 7-1 and 7-2). In contrast, cereal grains, soybean meal, rice bran and most vegetable oils are enriched in the omega-6 fatty acid, LA. For example, corn oil and soybean oil contain over 50% LA, and the fat in rice bran contains approximately 35% LA (Table 7-1
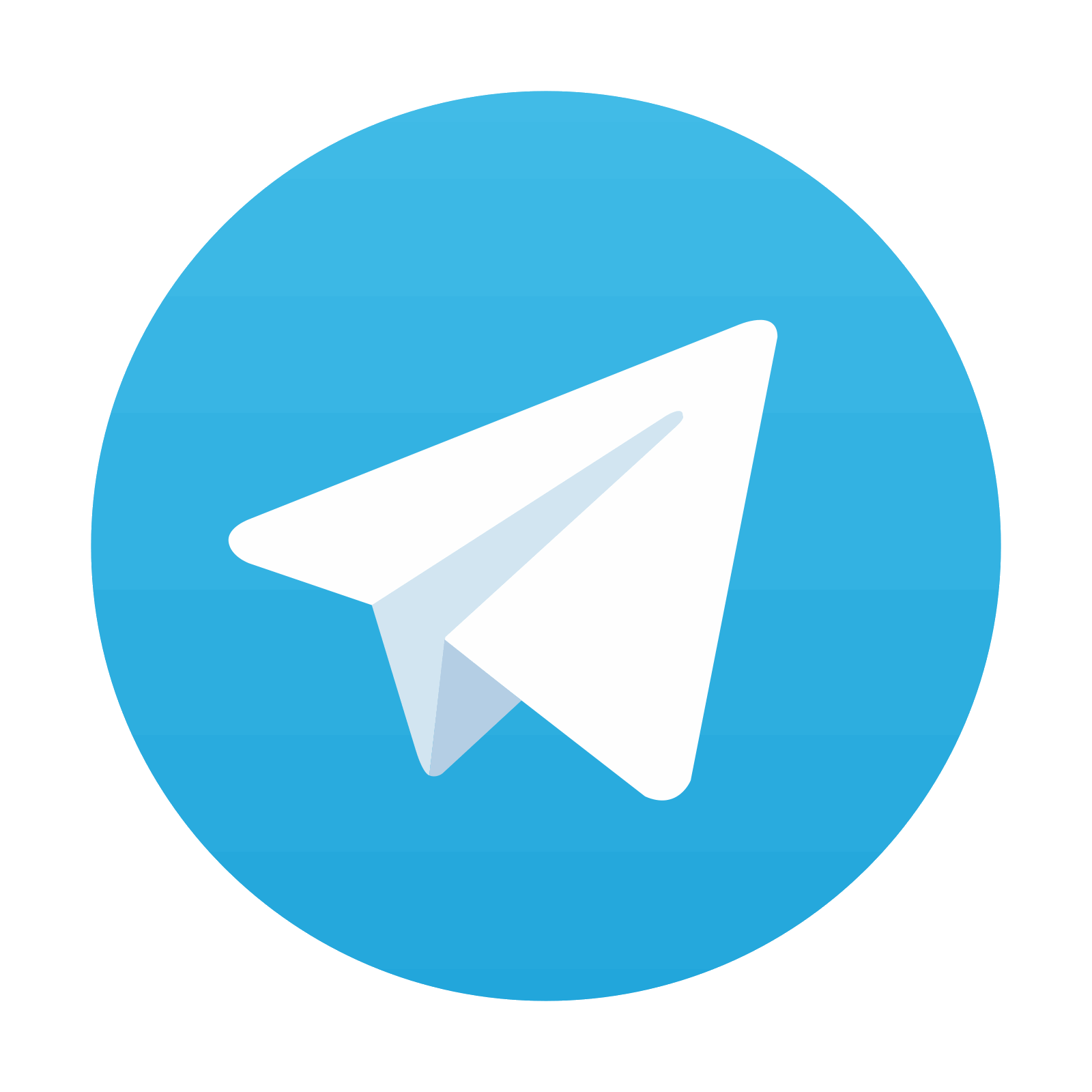
Stay updated, free articles. Join our Telegram channel
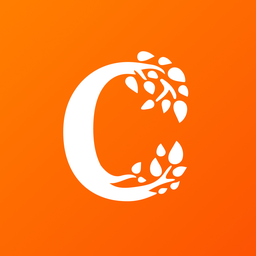
Full access? Get Clinical Tree
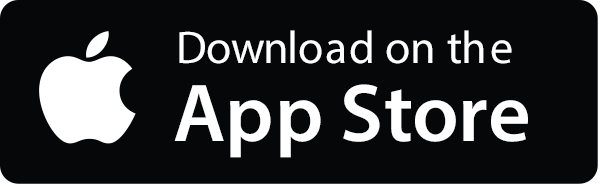
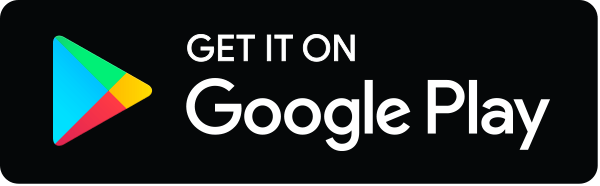