Chapter 11 Equine Vision
“Seeing”
Although a seemingly esoteric concept, it is critical to recognize that “seeing” is a perception and not a faithful rendition of the external world in all its factual detail. Indeed, the visual system of all species that have been studied to date takes great liberties with the data it receives from the external environment and uses a number of shortcuts to more rapidly process the image into information that can be used to guide behaviors. One such shortcut is to “see” things in context rather than as they truly are. Both horses and humans have been shown to be subject to the Ponzo illusion in which two horizontal lines of the same size are perceived by the brain to be two different sizes if they are presented in the context of a background set of lines similar to a converging pair of railroad tracks (Fig. 11-1).1 Similarly, it is quite probable that horses also perceive light and dark in relative rather than absolute terms, potentially explaining certain behaviors such as resistance to crossing certain visual barriers or entering trailers or stalls, etc. (Fig. 11-2). In general, illusions such as these offer insight into how the brain processes images; objects in the environment may not be seen as they really are but in context with their surroundings.
The eye is often compared to a camera, possessing optical elements (cornea and lens) capable of changing focus (lens) and an adjustable diaphragm (iris) that assists in controlling the amount of light that passes to the photosensitive layer (retina) that captures the image. This represents only the initial process of vision, however, as there is active manipulation of the image captured by the photoreceptors, both within the neural retina and subsequently by the central nervous system. Seeing is a complex process that depends on (1) light from the outside world entering the eye and being properly focused onto the retina, (2) the retina changing light energy into chemical energy and subsequently into electrical energy, (3) segregation of these responses into various categories (brightness, motion, location, etc.) and transmission of this information to the brain, (4) the brain processing this information to make it useful, and (5) selection of the relevant portions of the image for attention and further action (Fig. 11-3).2
The first two steps are comparable to a camera, but the act of seeing is not simply faithfully recording every feature of a scene as a camera would do, because that would quickly overwhelm the brain with huge amounts of information that would be irrelevant to the horse’s lifestyle or survival. Instead the brain must decipher the relevant aspects of an image from the billions of photons that interact with more than 100 million photoreceptors in each eye every second. In reality, the optic nerves are flooding the brain with massive amounts of information, much like a large telephone cable serving a major metropolitan area simultaneously carries millions of phone conversations. The brain has coped with this information overload by evolving to not consciously “listen” to all these “conversations” at the same time, but instead to categorize them into specific “topics,” which are channeled to specific areas of the brain for further processing.3–6 Unlike a camera, the brain compares this input with previous images (emphasizing changing aspects of the image), images from the other eye, and input from other senses such as hearing, smell, and touch. Once this is completed, only the information relevant to the task at hand or the horse’s survival rises to the level of conscious attention (Fig. 11-4). Interference with the process by which an object is determined to merit visual attention, even though it is clearly visible, is a common cause of accidents such as running into fences, the horse hitting its head on stall doors, throwing riders, etc. The act of seeing depends not only on the function and health of the eye but also on the cognitive processes in the brain that decide what information merits conscious attention and what is to remain subconscious or be discarded.
Extensive research into the visual pathways of numerous species, including the horse, has revealed that the information carried from the eye is packaged into many discrete topics such as an object’s brightness and size, location of its edges, its “internal” features such as texture and contrast, direction of movement, velocity, overall orientation as represented on the retinal surface, shape, color, and many other parameters. The most critical aspect of vision, however, is the ability to identify an object (a wolf, for example) as separate from its surroundings (dense vegetation). Because this distinction is so important for survival, animals (including humans) with normal vision can “see” an object if it differs sufficiently from its surroundings in any one of six different aspects: luminance (brightness), motion, depth, texture (which is related to visual acuity), orientation, or color (Fig. 11-5).6 On the basis of their luminance, motion, depth, and texture, objects are roughly differentiated equally well, but separations based on color are less easily made.3–6 In this chapter, we describe these fundamental ways of seeing objects as different from their surroundings, but in reality, a complete description of how well a horse sees requires not only an understanding of each of these facets of vision but also an understanding of how the brain integrates the constituent parts into a unified perception of the world. Unfortunately, the horse’s higher visual pathways remain poorly understood, so our understanding of equine visual perception has significant gaps.
Luminance and the Perception of Light
The ability to perceive light is the most basic aspect of vision. Although some birds are able to see into the ultraviolet spectrum and some snakes use infrared wavelengths to hunt (using specific nonocular receptors located in pits), the eyes of most mammals, including horses and humans, are capable of detecting photons located in only a tiny portion of the electromagnetic spectrum—typically between 380 and 760 nm (Fig. 11-6).7–10 Even in this visible spectrum, not all photons are detected equally well, and the specific photoreceptors which are optimally tuned to specific wavelengths are not uniformly distributed across the retina. For these reasons, light perception depends not only on the number of photons “raining” onto the retina but also on the spectral composition of the light entering the eye and the sensitivity of the eye’s photoreceptors to particular wavelengths.3,4 In other words, the ability to detect light in the visible spectrum depends on the type, density, and spatial distribution of photoreceptors that are present, and this will vary from species to species and even individual to individual.
The term luminance (sometimes called value by artists) is the perceived lightness of an object.6,11 Luminance is not purely a physical phenomenon, because the perception of dim-appearing “blue” light and bright-appearing “yellow” light may result from exactly the same number of photons of these different wavelengths striking the retina. Luminance also depends on how sensitive the rod and cone retinal photoreceptors are to the wavelength of light striking the retina.3,11 In bright light, where the cone photoreceptors are most active, the equine eye is generally more sensitive to wavelengths in the yellow end of the visible spectrum. Light in the green range of the visible spectrum is detected somewhat less effectively, followed by lesser sensitivity to red, and even less sensitivity to wavelengths in the blue portion of the visible spectrum. In dim light, where the rod photoreceptors are most active, light in the green-blue end of the spectrum is better perceived (peak sensitivity of rhodopsin is approximately 500 nm). Therefore, a yellow leaf may appear brighter than a green leaf under bright-light viewing conditions, which favor the use of cone photoreceptors that are most sensitive to yellow (peak sensitivity is approximately 545 nm). Under dim-lighting conditions, when the rod photoreceptors are most active, the green leaf may now appear brighter or more luminous than the yellow one. This phenomenon, called the Purkinje shift, also explains why blue objects appear lighter and red ones appear darker in twilight versus daylight (Fig. 11-7).12 Another way of appreciating the difference between luminance and color is to remove all the color information with a computer program and look at a scene in shades of gray.11 When this is done, it becomes obvious that many objects can be differentiated on the basis of their luminance alone; color is a less important clue (Fig. 11-8). Luminance is important because the perception of depth, three-dimensionality, movement (or lack thereof), and spatial organization are all carried by a part of the visual system that responds only to luminance differences and is insensitive to color.4,5,11,13
A major challenge confronting horses is to adapt to light intensities that vary from the dimmest star to bright sunlight on snow, a factor of as much as 1 to 40 billion.14,15 As noted previously, humans, and undoubtedly horses, are largely unaware of this enormous variation in light intensity, principally because objects are perceived in the context of their surroundings and not in absolute terms. Several mechanisms are used to adjust to this wide range of illumination, one of which is the previously mentioned Purkinje shift wherein a duplex retina (possessing both rods and cones) uses rod photoreceptors for dim light and cones for bright light.3,14,16–18 Rod photoreceptors can reliably respond to a single photon of light and are the primary receptors used when the light levels range from virtually complete darkness on an overcast moonless night to those found at dawn and twilight.14,19 By midmorning on a sunny day, the rods have become saturated by light, and in order for useful vision to be maintained, the eye must shift over to using primarily cones. This is analogous to a photographer switching from a fast-speed, “coarse-grain” black-and-white film designed for obtaining less detailed grayscale images in low light to a slow-speed, “fine-grain” film designed to produce highly detailed color images in bright light. However, as the eye shifts from rod to cone photoreceptors, what is lost in terms of sensitivity to light is replaced by improved color vision and visual acuity.
In addition to switching between rod and cone photoreceptors, there are several other mechanisms for adjusting to the wide range of lighting intensities that occur in the real world. Changing pupil size can rapidly alter the amount of light that reaches the retina, but this mechanism limits the range of light intensities only by about 10-fold in humans14 and probably only by a few fold more in horses. The horse eye is superior to the human in this regard because its pupil diameter has a greater dynamic range.20 Another relatively rapid but more robust mechanism involves changing neural processing by the retinal bipolar and ganglion cells. These cells can sum the light signals from a group of photoreceptors (spatial summation) or over time (temporal summation), and the retina can facilitate adaptation over a range of about 1000-fold.14 The large size of the horse’s eye serves to facilitate this process because it allows the light signal from neighboring photoreceptors to be summated, thereby brightening the retinal image. The summation process would potentially result in loss of the ability of the eye to resolve detail, but the large eye of the horse compensates for this by providing for a greater number of photoreceptors.21 The major but slower mechanism for adjusting to varying light intensity is to chemically alter the sensitivity of the photoreceptors to light.3,22 Light chemically dissociates the retinal photopigments into their constituent parts, and this depletion (photoreceptor bleaching) leads to a proportional increase in the amount of light required to trigger a response by the photoreceptor.3,14,22 Conversely, when an animal is placed in darkness, the photopigment is reconstituted into its active form, and the eye becomes more sensitive to light. This light and dark adaptation occurs over a period of minutes (5 minutes for cones and 20 to 30 minutes for rods) and can easily be appreciated by simply walking into and out of a dark barn on a bright, sunny day.3,14 In humans these photochemical changes can provide a range of adaptation to light intensity that varies on the order of 100 million–fold.14 Hence, vision is maintained over an enormous range of light intensities by making relatively slow but massive changes in photoreceptor sensitivity and then fine tuning the intensity by faster alterations in pupil size and retinal processing.
Other Ocular Adaptations
In addition to these mechanisms, horses have a number of other adaptations that improve vision in dim light that humans do not (Fig. 11-9). The horse has one of the largest eyes among the terrestrial vertebrates, and this allows more light to enter the eye through a large cornea and the pupil.23,24 Admission of light to the eye is further enhanced by horizontal elongation of the cornea and pupil25,26 and by the equine pupil’s ability to dilate to an area six times larger than that of a human pupil (3 to 3.5 times greater than cat’s or dog’s).20,27,28 An elongated slit-like pupil is common in species that are active at night and daytime, because in bright light this shape closes more completely than a round pupil, thereby better protecting a highly light-sensitive retina from very bright light.29,30 Because the pupil is located in front of the nodal point of the optical system of the eye, the horizontally elongated, roughly rectangular shape of the horse’s pupil in daylight also provides it with a wider view of the horizon than it would obtain with a circular pupil with identical surface area.29 This allows the horse to scan the visual horizon while at the same time reducing the variations in luminosity the retina must cope with between the usually brighter sky and the usually darker ground. The equine version of the rod photopigment, rhodopsin, also differs somewhat from that of humans,31 and like that of dogs and other species adapted for vision in dim light, it may continue to increase in sensitivity to light for a longer period when the animal is placed in the dark.32–34 Indeed, it may take more than 30 minutes or more for a horse to fully adapt to dim light conditions.18
The horse’s vision in dim light is also improved by a large, superiorly located, roughly triangular reflective tapetum lucidum that provides the photoreceptors a second chance to capture each photon (Fig. 11-10).35–42 However, this improved ability to capture light comes at the price of reducing the potential visual acuity of the eye, because the retina is unable to determine whether the photon hit the photoreceptor on the first pass or was reflected there from a slightly different point in space. In horses, the tapetal reflection is accomplished by the regular spacing of uniform-diameter collagen fibrils in the choroid.36,39,41 The color of the tapetum as seen with an ophthalmoscope is the result of the physical interaction of light with the fibrous tapetum, not the result of colored pigments in the tapetum.36,39,41 Yellow or green tapetal reflections represent regions where these fibrils are more numerous, whereas deep blue to purple reflections represent thinner regions of the tapetum. In contrast to the fibrous tapetum characteristic of most mammalian herbivores, the tapetum of carnivores is typically composed of cellular elements.35,41–43 Although the equine tapetum is not as efficient a reflector as the cellular tapetum of some nocturnal carnivores such as the cat (which can reflect up to 130 times more light than the human fundus),42 it is undoubtedly superior to the human eye, which lacks a tapetum altogether. The modification of different constituents (collagen, cells, or even crystals in some species) to create a reflective layer in the fundus and thereby improve vision in dim light suggests that the tapetum has evolved separately on a number of occasions and represents an evolutionary “arms race” between various predator and prey combinations in which each is seeking a distinct survival advantage over the other.24,26,35,37–41
The presence of a tapetum suggests that the horse’s lower limit for vision in dim light is much less than that for humans. It is not as low as that for cats, however, (5.5 to 7 times less than that for humans44) and perhaps that of certain other predators with a bright reflective tapetum. A recent behavioral study that required horses to discriminate between circles and triangles in a variety of dim-light circumstances in order to receive a food reward found that horses could readily make these kind of visual discriminations and navigate a room with obstacles at light levels that were so low a dark-adapted human could not see at all.45 They were fully capable of discriminating between shapes at light levels approximating those of moonlight, starlight on a moonless night, or under cloud cover at night. In fact, only when light levels approximated those of a dense forest with minimal visible sky at nighttime, or a dark enclosure devoid of almost all light, did they lose their ability to make visual discriminations. Even in this very dim lighting circumstance, however, the horses were still able walk around the testing area, locate a feed bowl, and walk through a small testing station set of stocks without bumping into objects.45 These experimental observations are consistent with anecdotal observations that wild mustangs are able to run at full gallop over rough terrain while negotiating sagebrush, rocks, hills, and gullies with only starlight as the light source.45
Although the equine visual system appears to have evolved to function well in dim-lighting circumstances, it also has several features that improve vision in bright light, suggesting that the horse is adapted for an arrhythmic photic lifestyle. For example, the nuclear region of the equine lens contains yellow pigments (Fig. 11-11),46 as do human lenses and those of other highly diurnal species such as squirrels.47,48 These pigments filter out shorter (blue) wavelengths of light, much as yellow-tinted sunglasses do for humans, thereby reducing glare in bright light and improving the contrast of some objects against their background, since blue wavelengths of light are scattered 16 times more easily than red wavelengths.47,48 This pigment also filters out higher-energy rays that are more likely to damage the retina, thereby protecting the delicate photoreceptors of the ventral retina in bright daylight.47,48 The large corpora nigra on the central superior border of the iris also improves vision in bright light by augmenting pupillary constriction and acting as an internal visor that decreases the amount of light entering the eye from the superior visual field (where the sun is located), further reducing glare and improving vision in bright light. The much smaller corpora nigra on the inferior pupillary border may behave in a similar way to reduce the impact of light reflected off the ground. In fact, in very bright light, the horse’s pupil may constrict to such a degree that the superior corpora nigra apposes the border of the inferior iris, effectively dividing the pupil into two segments. The impact of this on the animal’s vision is unclear, but two pupils oriented in the same plane as the visual streak on the retina may improve the animal’s vision in bright light29 (see discussion of optical consequences of pupil shape). In a sense, the horse has evolved to be a visual generalist, with some degree of vision under all lighting circumstances, unlike humans who tend to function best in bright light and cats who function well in dim light.
Detecting Motion
Detecting motion is another fundamental component of vision with great biological importance. Moving objects are more likely to demand some type of response on the part of the horse than stationary ones, and horses, like humans, detect moving objects more easily than stationary ones. This is especially true for objects in the horse’s peripheral visual field, which has a visual acuity so low that it may permit only movement and brightness, rather than discrete objects, to be seen.24 This poor peripheral visual acuity coupled with a prey mentality may explain why horses often shy from even innocuous objects that move in their peripheral visual field. Although the peripheral retina has often been described as being best at detecting motion, it is inaccurate to infer that animals with large peripheral visual fields are better at motion detection than those with better-developed central visual areas such as a fovea. The peripheral retina does have a greater number of the ganglion cell subtypes devoted to motion detection,49,50 but the central retina has more densely packed photoreceptors. Because of this higher resolution, it actually has a much greater ability to detect motion than does the peripheral retina. In one study, the more densely packed human fovea had a 10- to 12-fold lower threshold for detecting motion than that of a cat.51,52 Horses have significantly better visual acuity than cats, so it is likely they are better at detecting motion than cats but not quite as good at it as humans. It remains to be determined, however, whether there are differences between the peripheral retina and central retina of horses that would render their peripheral vision more sensitive to objects that are moving at specific speeds, in certain directions, or possess features that make them more “attention grabbing” for some reason.
In real life, detecting motion can be quite complex because movement may occur on part of the object, the horse, or both. Motion detection is most easily accomplished if the horse itself is stationary and the object is moving. In that circumstance, any change in the position of the object on the retina generally signals that it is moving. If both the horse and the object are moving, however, motion detection becomes quite complicated because the animal must now closely coordinate the movements of its eyes, head, neck, and entire body to prevent the retinal image from “bouncing” in such a jerky manner (like the image captured on a video camera when the operator is running) that the animal would be functionally blind.14 When a horse is trotting, some compensatory mechanisms can be seen at work: the head and therefore the eyes bob only slightly because of a series of reflexive counterbalancing motions by the body and neck (Fig. 11-12).
Because maintaining vision while the horse or an object is moving is so critical to the animal’s survival, several different systems have evolved to stabilize images on the retina. Virtually all mammals, including horses, use a stabilization (fixation) system, a vestibulo-ocular reflex system, and an optokinetic system to stabilize images on the retina.53–55 An additional set of more complicated eye movements—saccades, smooth pursuit movements, and vergence eye movements—are found in species that have forward-looking eyes, comparatively smaller fields of view, relatively well-developed extraocular muscles, a fovea (or at least a localized central region with heightened photoreceptor density such as the area centralis in cats), and improved binocular vision.53–55 These movements allow the region of the retina with the greatest visual acuity to be aimed at and track an object, especially while both the animal and the object of interest in the environment are moving.
Most of a human’s perception of vision (and presumably that of a horse) occurs when we fixate our gaze. The fixation system (1) attempts to keep objects of interest in a relatively stable position on the area of the retina with the greatest visual acuity and (2) helps prevent the image from being so stable on the retina that the retina undergoes sensory adaptation and the image no longer becomes visible. Although these two aims appear to be at odds with each other, it is important to remember that because the brain takes shortcuts and emphasizes changes in an object rather than its true physical nature, perfect stabilization of an object on the retina would soon result in it being no longer apparent (Fig. 11-13). Hence, the fixation system consists of both eye movements that keep the area of greatest visual acuity directed at an object and a set of very small, involuntary eye movements that prevent the image from being perfectly stable on the retina. The precision of these movements means that the fixation system works well only if the object is completely still or moving very slowly. It tends to quickly become inoperable if the object, the horse, or both are rapidly moving.
Two even faster reflexive eye movement systems also participate in retinal image stabilization. These pathways work to cancel out motion of an object across the retina when the horse itself is moving (the vestibulo-ocular reflex or “doll’s eye” reflex) or when object is moving across the horse’s visual field and the horse is stationary (optokinetic nystagmus). The differences between these two methods of stabilizing an image can be appreciated by holding up one finger at arm’s length. The vestibulo-ocular reflex can be simulated by rotating your head side to side while at the same time having both eyes remain focused on your finger. You will notice that although your head is moving, the image of your finger remains relatively stable. This is because the semicircular canals of the vestibular system detect movement of the head, and this information can be used to rapidly direct the eyes to move to counteract the anticipated effects of that motion and keep the object in focus. In contrast, the optokinetic system can be simulated in this example by keeping your head stationary and moving your finger rapidly from side to side. In this situation, the image of your finger is much less stable and appears blurred. Here the stimulus for perceiving motion is not movement in the semicircular canals, but the retina detecting an image “slipping” very quickly across the visual field. Because the image must move before it can be detected, and only then can this information be used to redirect the eyes, the brain is always one step behind where the finger was in space, so the image appears blurred. Although the vestibulo-ocular reflex is better at stabilizing an image on the retina as the head is accelerating or decelerating, if the head begins to move at the same speed, the fluid in the vestibular canals soon begins moving at or near the same rate as the head and can no longer be used to direct the compensatory eye movements. The image, however, is still slipping across large regions of the retina, and now the phylogenetically old optokinetic nystagmus system takes over, and the brain switches over to using this visual stimulus to continue to direct the eye movements in an attempt to keep the image stationary on the retina.53–55
It is also possible to trick the brain into activating the optokinetic nystagmus system by keeping the animal stationary and making the surrounding environment appear to rotate around it. The latter trick, consisting of a rotating drum or projected series of bars (Fig. 11-14), has been used to estimate an animal’s visual acuity because this type of nystagmus only occurs if the animal is actually able to distinguish the object in question (usually a series of light and dark bands) as separate from the background.43 The limit of visual resolution in this case is determined by varying the width of the lines on the drum (or the speed in which the drum rotates) and establishing the maximum line width (or speed) at which the optokinetic response is no longer initiated. In effect, when the nystagmus disappears, the animal no longer sees individual light and dark bars, and the image appears uniformly grey. The spacing between the bars at which this occurs can be used to calculate the animal’s visual acuity or ability to resolve fine details. This is a less than ideal method. The stimulus for the optokinetic reflex is the image “slipping” on large regions of the retina, rather than the fine details of the object as viewed by the visual streak or fovea.53–55 Because estimates of visual acuity obtained in this manner are not limited to the regions of the retina responsible for the greatest visual acuity (visual streak) but include larger parts of the retina that have lesser acuity, this method may result in underestimating visual acuity. Nevertheless, the vestibulo-ocular reflex and the optokinetic system can be thought of as a single system that uses two different sensory signals, vestibular and visual, to help solve the same problem: maintaining retinal image stability during self-rotation and movement.
In addition to these three involuntary stabilizing reflexes, many species that have been investigated (and probably the horse) also have voluntary eye movements such as saccades and smooth pursuit movements that allow the animal to voluntarily shift its gaze to keep images on important regions of the retina.53–55 Saccades are rapid (0.02 to 0.1 second) voluntary movements that quickly shift the eyes to keep a moving or new image on the area of the retina with the greatest visual acuity. These “jumping-ahead” movements may be quite large, covering up to 100 degrees in humans.54 When the head is stationary, some species such as the rabbit and goldfish do not make saccades for all practical purposes.54 These species have little need to do so because their laterally placed eyes already provide them with a large field of view, and their horizontally oriented visual streak allows them to scan the horizon without moving the globe. When a rabbit (and under certain conditions, a horse) makes a voluntary head movement, a saccade is initiated simultaneously with, or just before, the head movement in the direction the head is turning.54 This causes the gaze to jump ahead, and while the head is moving, the eye holds its new position by means of the vestibulo-ocular reflex until the head catches up and the gaze is again properly aligned.54 Humans and animals with a central area of particularly acute vision (area centralis), such as dogs and cats, can also make voluntary saccadic eye movements without moving the head. Although the horse also has laterally placed eyes and a large field of view, it appears to be capable of making saccades without moving its head, especially when it is apprehensive, but does not seem to make these movements as often as animals with more frontally located eyes.
Because the eye rapidly “skips ahead” during a saccade, there is no time for the retina to process the intervening visual information or for the brain to correct or refine the movement during the shift. In effect, the eye is functionally blind during a saccade, and the target may be slightly undershot or overshot.53–55 If the eye is shifting a large distance, the initial saccade moves the eye about 90% of the distance, and one or more smaller corrective saccades make up the remainder. The “smearing” of the image on the retina during a saccade is generally not consciously appreciated, and even though the eye has moved a large distance, the external world is perceived to be stable.53–55 This is another way the eye differs from a camera; when a camera moves, the external world would also appear to move. Once the image stabilizes on the retina at the end of a saccade, the new information overpowers that which may have started during the saccade, and higher visual pathways “backward mask” the old information, thereby smoothing the image and rendering the animal unaware of the rapid, large shift that occurred.54 It is tempting to speculate that because the horse has a large field of view, it needs to make fewer saccades, and this improves its vision (at least to a minor degree) by virtue of a reduction in the image smearing that occurs during these movements.
In many species, once the image has been captured on the visual streak by a saccade and any small catch-up saccades have been made, relatively voluntary, smooth-pursuit eye movements track a moving object so that its image remains on the retinal area with the greatest visual acuity (fovea or area centralis).53–55 Like the saccadic system, smooth pursuit evolved with the development of retinal areas of greater resolution and is most well developed in species equipped with this type of retinal architecture. Under normal conditions, the stimulus for smooth pursuit is similar to that for the optokinetic system—for example, movement of the image across the retina (retinal slip).54,55 Smooth pursuit differs in that the movement of the object is the point of interest. With the optokinetic system, the stimulus is much larger, and the goal is to follow the background, which is moving in the opposite direction, as the point of interest. Smooth-pursuit movements are driven by images that stimulate the fovea or area centralis and are usually small and relatively slow, whereas the slow phases of the optokinetic system are induced by large patterns that stimulate large areas of the retina.53 To be effective, smooth-pursuit eye movements must override not only the fixation maintenance system, which tries to keep the eyes fixated on the same point in space, but also the optokinetic system, which tries to follow the massively slipping background images instead of the target.54 If the horse’s head is also moving, the vestibulo-ocular reflex needs to be cancelled out if smooth-pursuit movements are to allow the image to be examined more closely.54 In all these circumstances, the central visual processing system of the horse must decide whether to consciously pay attention to the background or the more focal point of interest, and which system to override to achieve this goal.
Stationary objects are also viewed with smooth-pursuit eye movements, because there is always slight motion of the image on the retina as the muscles of the head, neck, and body attempt to maintain a steady posture.14,53–55 Because smooth-pursuit eye movements track an image by using “retinal slip,” the image on the retina is always moving to some degree. This is another way in which the visual system differs from a camera: in effect, it requires some degree of movement to function properly. Although the image of the object being observed may seem to be stationary, this is purely a perceptual phenomenon and is the result of central visual pathway processing of the image. Animals with visual streaks (such as horses) typically do not show smooth-pursuit movements when an object moves horizontally along the direction of the streak, but they appear to do so when an object moves vertically across the streak.14
Saccadic and smooth-pursuit movements result in the two eyes moving equally in a synchronous (conjugate) fashion, and the viewing angle between the two eyes remains the same. Typically, these movements are elicited when the object of interest is moving from side to side or up and down. If the object of interest is moving toward or away from the viewer, convergent or divergent “vergence” eye movements, respectively, are used to change the viewing angle between the two eyes to keep the object in proper focus. Convergence is also usually accompanied by accommodation (adjusting the focal power of the lens so that near objects are seen more clearly) and miosis that optically increases the animal’s depth of field, thereby enhancing depth perception and visualization of near objects.56 These three responses—convergence, accommodation, and miosis—are neurologically distinct, but because they generally occur simultaneously, they are referred to as the near response.56 Vergence eye movements are typically fairly slow (taking up to 1 second in humans),53 and as during a saccade, visual sensitivity is markedly reduced during the movement itself. The extent to which horses perform vergence eye movements has not been carefully investigated, but the relatively large area of binocular overlap and certain behaviors (see discussion of depth perception) suggest that they are readily capable of performing these types of movements. However, the comparably slower rate of pupillary constriction of horses versus that of humans suggests that horses may perform vergence movements more slowly than humans do.
The Perception of Depth
Visual Perspective and Field of View
The ability to differentiate objects on the basis of depth depends on a number of factors: the animal’s visual perspective, field of view, the difference between the images acquired when both eyes view the same object (binocular disparity), and higher processing by the brain to fuse these images into a novel image that allows for depth to be perceived. The horse’s visual perspective can vary greatly and depends to a large extent on whether the head is down and the animal is grazing, or the head is up and the animal is scanning the horizon. Furthermore, breed differences can result in the same environment being perceived quite differently. A field of tall grass may appear to be a dense, impenetrable brush to a Miniature horse but a wide-open savanna to a Clydesdale (Fig. 11-15).
The lateral position of the eyes in the skull affords the horse a wide panoramic view (Figs. 11-16 and 11-17),16,24,30 and the nasal extension of the retina further enhances the horse’s temporal peripheral visual field. On the basis of anatomic relationships, the horse is believed to have a total monocular visual field in the horizontal meridian (i.e., the portion of the horizon that can be seen by an eye when fixed on one point) of approximately 190 to 195 degrees, and up to 178 degrees in the vertical (superior to inferior) meridian. When the visual fields of the two eyes are combined, the total horizontal visual field is up to 350 degrees, and the horse has virtually a complete sphere of vision around its body, with only a few minor “blind spots.”16,24,30 These blind spots are small and located superior and perpendicular to the forehead, directly below the nose, in a small oval region in the superior visual field where light strikes the optic nerve itself, and the width of the animal’s head directly behind it. Clearly, this extensive visual field makes it very difficult for a predator or human handler to sneak up on a horse.
The visual fields of the two eyes overlap anteriorly and below the nose for 55 to 65 degrees, although some authors suggest the overlap may be as great as 70 to 80 degrees.16 This degree of binocular overlap rivals or exceeds that of domestic dogs, which ranges from 30 to 60 degrees, depending on breed.24,57–59 This would seem to challenge the generally accepted view that predators have binocular vision superior to that of prey species. In reality, however, depth perception plays a significant role in breaking the camouflage of an object (e.g., a wolf) against its background, and this would be of distinct advantage to prey species.60 As has been described for certain bird species, it is also possible the animal’s lifestyle and the extent of the binocular field do not necessarily predict the quality of its stereoscopic vision.61,62
Stereopsis (binocular depth perception) results when the two eyes view the world from slightly different vantage points, and the images from the overlapping visual fields are fused into one.60 If the relatively disparate images on the two retinas were not blended, viewing a scene with both eyes would simply result in double vision (diplopia), and depth perception would actually be impaired. The new information that stereopsis provides is as qualitatively important to the overall sense of vision as is the perception of motion, color, or contrast. The ability to perceive depth varies greatly between individual humans63,64 and cats,65 and although similar work has not been done with horses, some horses undoubtedly are better than others at detecting depth. How these differences may affect their usefulness for certain types of work is uncertain because a number of horses with one eye have been observed to still function well as jumpers or barrel racers, even though these tasks would seem to demand excellent depth perception. Also, several horses with only one functional eye have performed well in top-tier races such as the Kentucky Derby.66
Integration of binocular visual inputs is not the only means available to an animal to accomplish depth perception, however. As any artist can attest, animals and humans can appreciate depth with only one eye by using specific visual clues. These include relative brightness (brighter objects tend to be closer), size (larger objects are closer or objects progressively increasing in size are getting closer), contour and areas of light and shadows (the gradation in the intensity of a shadow on a curved object makes it appear to be projecting away from or toward the observer), object overlay (closer objects block distant ones), linear perspective (parallel lines like those of a railroad track converge onto a vanishing point at distance), aerial perspective (water vapor, dust, and smoke in the atmosphere make distant objects indistinct and relatively color-desaturated), density of optical texture (the fine details of the surface of an object become less apparent the farther away it is), relative velocity (near objects appear to move faster than distant ones), and motion parallax (objects at different distances appear to move at different speeds or in different directions, depending on the observer’s point of fixation (Fig. 11-18).60 There is good evidence that horses are able to use at least some of these static monocular clues to recognize depth in two-dimensional photographs, and it appears they are even susceptible to some of the pictorial visual illusions to which humans are susceptible.1,67 Because of their huge monocular visual fields, it is clearly advantageous for horses to be able to use monocular clues to estimate depth.
Nevertheless, two eyes are better than one, because the binocular threshold for depth perception in horses is five times better than the monocular threshold.68 Although the two eyes of horses are farther apart than those of humans (resulting in a greater relative disparity between the two retinal images and theoretically better depth perception), humans still have better depth perception than horses. From 2 meters away, humans can detect a few millimeters’ difference in depth, whereas from the same distance, horses can detect only a 9-cm difference (a 10- to 20-fold poorer performance).68 The latter value approximates that of cats69,70 and may have some biological relevance because it is also approximately the same difference in depth between grass in a pasture and the ground under it. The familiar behavior of a horse rotating its nose upward to better observe distant objects has been suggested to be a response to exploit improved depth perception, since binocular overlap is said to be oriented down the nose in horses (rather than straight ahead as it is in humans).16,24,71 Evaluation of the eye position and forward visual field of the horse, however, would suggest that they are still capable of binocular overlap when viewing objects with their noses down and their eyes directed forwards, as when riding “on the bit.”72
When stereopsis is combined with the perception of motion, horses and humans are able to make accurate judgments regarding the trajectories and distances of moving objects so as to allow them to be intercepted or avoided. A similar phenomenon occurs if the object is stationary and the horse is moving. If a fixed point of reference is present, it is easier to detect motion-in-depth than if the object is effectively by itself.73 For example, it is easier to detect the motion-in-depth of a baseball bouncing along the ground than it is to detect its motion-in-depth against a clear blue sky. Predators use stereopsis and stereomovement (the ability to detect motion-in-depth) to improve the accuracy of their attacks, but as discussed previously, these visual components are equally important to prey species such as the horse because they enhance their ability to detect the predator’s camouflage and avoid objects during their escape.60
The ability of humans with normal vision to detect motion-in-depth is 5 to 28 times poorer than their ability to detect a difference in depth if the object remains static (stereoacuity).73,74 At first, this is somewhat surprising because (1) objects that are moving from side to side (e.g., in the frontoparallel plane) are much easier to detect than stationary objects, and (2) each eye working alone is readily able to detect objects that are moving across the visual field from nasal to temporal and also moving away from or toward the eye.75 This phenomenon appears to be due at least in part to the fact that stereomotion detectors average the motion individually detected by the left and right eyes.75 Additionally, objects moving across the visual field stimulate a series of photoreceptors over more widely separated regions of the retina, whereas objects moving in depth stimulate regions of the retina that are much closer to each other. Finally, this difference is not so much because detection of motion-in-depth is so poor, but more so because stereoacuity is so good. Brain processing of the images from both eyes allows details of an object that are not visible to either eye alone to be detected (see discussion of hyperacuity).
The ability to perceive depth develops after birth and depends on images being presented to both eyes for comparison purposes. This ability goes through a critical developmental period, and kittens that have had the eyelids of just one eye sutured closed for a brief period (5 days) will experience substantial permanent deficits in binocular depth perception. This is especially true if the deprivation occurs between 35 and 45 days after birth.76 This developmental age may correlate with that of a newborn foal and suggests that if the vision in one eye is impaired by a corneal ulcer or the placement of a third eyelid flap during critical periods of maturation of the visual system, depth perception may fail to develop normally in that animal, despite subsequent resolution of the problem.
Visual Texture
All surfaces of an object have a tactile texture—how they feel when touched. They also have a visual correlate of this called visual texture, which for our purposes refers to the appearance of the surface’s texture. In other words, does the surface appear rough or smooth, homogenous or fragmented, full of fine details or uniform, composed of randomly arranged elements or elements that occur in a regular pattern, and so on. Visual texture can be a powerful mechanism for seeing an object as separate from other objects in the visual field (Fig. 11-19). Visual acuity and form perception are other critical elements in the horse’s ability to see objects as separate from their surroundings.
Visual Acuity
In general, visual acuity refers to the ability to see the details of an object separately and without blurring. It depends on (1) the optical properties of the eye, (2) the retina’s ability to detect and process images, and (3) the ability of higher visual pathways to process and interpret these images. Visual acuity can be evaluated in many ways, and at times, the methods and terminology can be quite confusing. Measurements of visual acuity may be based on the size of a letter on an eye chart, the angle the object’s image subtends on the retina (in seconds, minutes, or degrees of arc), or how close together an alternating pattern of black and white lines can be before they appear to be uniformly gray (the so-called grating acuity, which is measured in cycles per degree). All of these measurements are interrelated, and tables that allow one set of units to be compared with another have been created (Table 11-1).77
In humans, one of the most familiar methods of measuring visual acuity is to ask an observer to read a Snellen eye chart, which consists of rows of progressively smaller black letters on a white background (Fig. 11-20).78 The size of the letters between rows varies in a geometric fashion (0.1 log unit), and the smallest line that can be reliably discerned in this high-contrast setting is called the minimum resolvable or resolution acuity.78 There are a number of variations on the classical Snellen eye chart, and because not all letters are equally easy to read, other commonly used charts use only one letter (typically a “tumbling E” or “Landolt’s C”) and ask the observer to indicate the direction in which the letter is pointing (up, down, right, or left). The size of each letter in a row is based on the assumption that under these optimal but artificial conditions, normal human visual acuity is 1 minute of arc, so the width of the strokes forming the E or the gap in the C will subtend 1 minute of arc on the retina at a specified distance (e.g., 20 feet, 60 feet, 200 feet).78 The results of these tests are then typically recorded as 20/20, 20/40, 20/60, and so on—the numerator representing the distance the subject is from the target (chart), and the denominator representing the maximal distance from which a person with statistically normal vision could identify a letter this size. In other words, 20/200 vision means that the test subject can only make out the details of a letter from 20 feet away that a person with normal visual acuity could make out from 200 feet away.
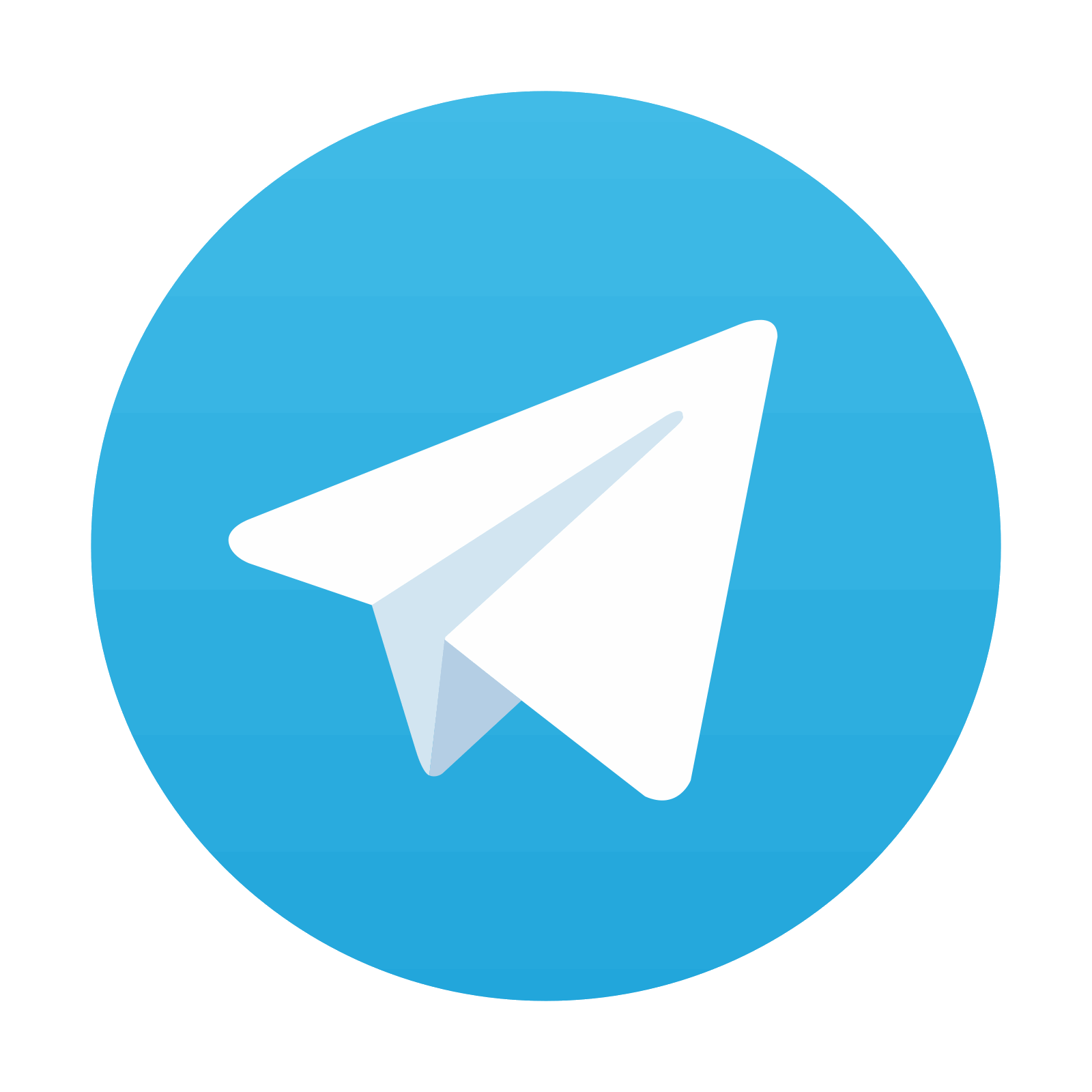
Stay updated, free articles. Join our Telegram channel
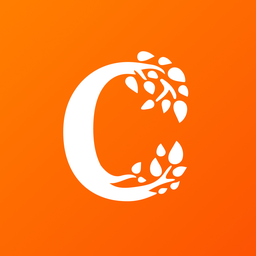
Full access? Get Clinical Tree
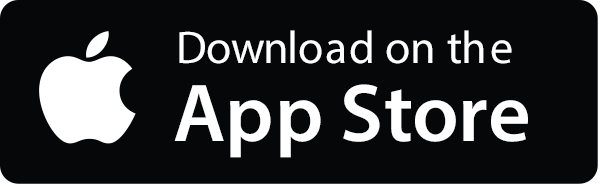
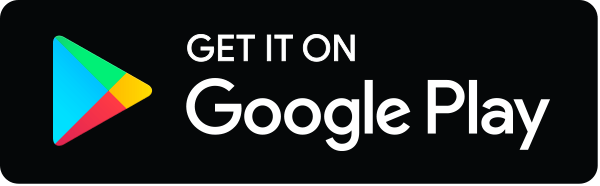