Pierangelo Renella1, Wyman W. Lai1, and Ashwin Prakash2 1 CHOC Children’s Heart Institute, Orange, CA; University of California, Irvine School of Medicine, Irvine, CA, USA 2 Boston Children’s Hospital; Harvard Medical School, Boston, MA, USA Central to the care of pediatric and adult patients with congenital heart disease (CHD) is the ability to accurately define cardiac and extracardiac vessel anatomy, along with cardiac chamber function, across a wide range of pathologies. In some cases, the presence of myocardial fibrosis may also be of interest. Infectious complications such as endocarditis may need to be ruled out. Imaging of the airway imaging may also be important in certain situations. In these scenarios, echocardiography (echo) can fall short. Modern congenital cardiologists have at their disposal multiple methods of cardiac imaging. These include the first‐line method of echo, as well as those which use magnetic resonance, computed tomography, and nuclear techniques. Each of these have inherent advantages and disadvantages that tend to complement each other, as will be discussed in this chapter. Multimodality cardiovascular imaging is the application of one or more of these techniques in a given patient. The decision to add one or more of these types of imaging studies to echo depends on several factors including the specific clinical questions, the necessary spatial and temporal resolution to adequately answer these questions, patient age and tolerability, radiation exposure, local expertise, and cost. The largest amount of relevant clinical information and optimal image quality should be sought, while minimizing risks of radiation and/or anesthesia exposure to the patient. In this manner, it is possible “personalize” the imaging approach to a specific patient for a specific need. The technical advances that eventually led to magnetic resonance imaging (MRI) of the human heart were pioneered in the early 1970s, with the first still images taken in the 1980s [1–3]. The 1990s brought the first moving images of the heart as well as the first attempts at magnetic resonance angiography (MRA) [4–6]. These advancements in cardiovascular MRI (CMR) increased the ability to evaluate intracardiac anatomy, chamber size and function, as well as delineate the extracardiac vasculature. Accurate noninvasive measurements of blood flow then followed [7,8]. Moreover, the ability to identify replacement myocardial fibrosis after myocardial infarction, nonischemic cardiomyopathy, and in the tissue characterization of intracardiac tumors, has further expanded the role of CMR [9–13]. In the last two decades, the value of CMR as an important diagnostic adjunct to echo in CHD has been recognized and its use has been recommended in various published guidelines [14–18]. A CMR image begins with a strong homogeneous magnetic field produced by a superconducting magnet, which serves to focus the precession of the numerous hydrogen atoms present in the body along the direction of that field [19]. The magnet producing the field is housed in a large cylindrical structure with a bore into which the patient is placed during image acquisition. With the protons of the hydrogen atoms aligned along the field, radiofrequency (RF) energy is then briefly applied to temporarily perturb these protons out of alignment. When the RF pulse is turned off, the protons realign with the direction of the strong static magnetic field (a process termed relaxation) and release RF energy [20]. This energy is detected by special antennas and the signal is forwarded to a computer processor. Fourier transformation is then employed to create image data. This is a similar mathematical process to that used in Doppler echo to generate image data from ultrasound. CMR images can be 2D or 3D, and either static or in‐motion (time‐resolved cine imaging) [21]. This process is quite different from the one utilized during cardiac computed tomography (CCT), which is typically faster, but relies on ionizing radiation to create images [22]. Although echo remains the first‐line diagnostic modality in children and adults with CHD, the comprehensive nature of CMR makes it indispensable, particularly in situations where echo is not adequate. These include imaging of older patients, those with larger body size, patients who have undergone cardiac surgery, and in the evaluation of the extracardiac vasculature (distal branch pulmonary arteries, pulmonary veins, and the entire thoracic aorta) [16,23,24]. Thus, CMR is particularly important in preoperative and preprocedural planning in the setting of complex CHD. Further, CMR has become an essential modality for the assessment of valvular dysfunction (e.g., quantification of regurgitation fraction), measurements of regional blood flow (e.g., differential pulmonary arterial and venous blood flow), myocardial properties (e.g., diffuse myocardial fibrosis, edema, iron concentration), and cardiac tumors. Importantly, as noted, the additional information can be obtained without exposing the patient to ionizing radiation [25]. This benefit is particularly advantageous in younger children, pregnant women, and in patients who require lifelong follow‐up imaging [16]. As compared with echo, CMR is a cross‐sectional imaging modality offering a large field of view and clear anatomic reference points to extracardiac landmarks such as the sternum, spine, and airways. The constraint of acoustic windows limits echo visualization of structures adjacent to the air‐filled lungs, scar tissue, subcutaneous fat, and the ribs. These may make the display of proper on‐axis four‐chamber and short‐axis views of the left ventricle (LV), right ventricle (RV), and heart valves challenging for echo. The 3D nature of CMR images allows for the display of true cross‐sectional electrocardiogram (ECG) gated cine images, and thus more accurate measurement of cardiovascular structures [26,27]. More recently, the advent of 3D cine CMR has further expanded the ability to reformat imaging planes in any arbitrary plane, independent of image acquisition plane. Of note, CCT also allows for multiplanar ECG‐gated cine imaging and 3D angiographic images (see later), but at the cost of ionizing radiation exposure to the patient [28,29]. The main drawbacks of CMR include lack of portability and relatively limited technologist and physician expertise as compared with echo and CCT. A typical CMR study lasts approximately 45–60 minutes, thus limiting its use in young children (less than 8 years old) and in patients with developmental delay or significant musculoskeletal pathology [30,31]. In these cases, sedation or anesthesia may be required to complete the imaging protocol. In contrast, echo and CCT are relatively faster imaging techniques, which may obviate the need for sedation in certain cases [32]. Due to the requirement for ECG gating, routine cine CMR imaging is sensitive to ectopy and arrhythmia. However, newer “self‐gating” techniques may eventually be able to overcome this limitation [33,34]. CMR is the only cardiac imaging modality with a contraindication for patients with cardiac implantable electronic devices (CIEDs) [21,35]. However, over the past decade, the development of MRI‐compatible pacemakers and experience of scanning patients with CIEDs have increased such that, under specific protocols, CMR can be performed safely [36]. As noted earlier, the complete assessment of intracardiac segmental anatomy is possible solely by echo in the majority of cases. However, when echo cannot delineate essential aspects of cardiovascular anatomy, CMR can be useful to confirm or fill in particular anatomic details such as: visceroatrial situs, atrial appendage anatomy, atrioventricular and ventriculoarterial connections, ventricular morphology and situs (D‐ or L‐loop), great artery anatomy, and the 3D spatial relationship of outflow tracts to the great arteries [37,38]. With the addition of MRA, complete and accurate visualization of the extracardiac thoracic vasculature is possible, and may obviate the need for invasive cardiac catheterization [39–42]. Echo may not always completely visualize the extracardiac vasculature. Thus, MRA can be particularly helpful in the diagnosis and pre‐ and postoperative management of aortic coarctation, branch pulmonary artery hypoplasia, and totally anomalous pulmonary venous connection, for example [40,43–46]. CCT can provide similar information more quickly, but at the cost of radiation exposure [47,48]. For this reason, MRA is better suited for the serial follow‐up of extracardiac vasculature after surgical repair (Figure 47.1). Cardiovascular MRI has been recognized as an important adjunctive imaging modality to echo and CCT in the assessment of valvular heart disease in children and adults with CHD [18,49–51]. Cine images with high spatial resolution, along with the ability to image in any plane in 3D space, allow for high‐quality on‐axis leaflet and valvular apparatus imaging [49]. Both forward and regurgitant flow across all four valves may be obtained using phase contrast MRI [52], as described in more detail later in this chapter. Post‐stenotic and regurgitant jets can be visualized and analyzed semiquantitatively on cine and phase contrast MRI images, albeit with lower temporal resolution than echo Doppler [52–54]. The most useful information about valvular function is derived from evaluation of the regurgitation fraction, which is calculated as the regurgitant volume divided by the total forward volume across a valve. A salient example with respect to CHD is in the case of repaired tetralogy of Fallot with residual pulmonary valve regurgitation leading to RV enlargement and dysfunction [55]. Another important clinical scenario is bicuspid aortic valve with the potential for aortic stenosis, regurgitation, and/or aortic dilation. CMR has been shown to provide accurate and comprehensive information about leaflet and commissure morphology, reproducible quantification of regurgitation volume and fraction, as well as 3D assessment of the entire thoracic aorta in all age groups, as shown in detail later [56–58]. The peak aortic valve flow velocity can be converted to a valve gradient via the modified Bernoulli equation. This gradient may be used to grade the degree of stenosis. However, due to technical challenges with the phase contrast MRI sequence, peak velocity calculated on CMR typically underestimates the measurement by echo [59]. Assessment of valve flow by CCT is currently not available. Figure 47.1 Comprehensive MRA evaluation of a patient with Fontan circulation (chest and abdominal coverage). (a–c) 3D volume renderings were reformatted from the steady‐state MRA along with colorization of the systemic arteries (red), systemic veins/Glenn/Fontan/branch pulmonary arteries (blue), and the portal venous system (fuchsia). Cardiovascular MRI is the clinical gold standard modality for the complete, accurate, and reproducible evaluation of volumes, function, and mass of both the LV and RV [17,18,60]. The relatively high spatial and temporal resolution of CMR images allows for good endo‐ and epicardial border visualization with no limitations due to acoustic windows, body size, cardiac position, or thoracic scar tissue [61]. Volumetric and functional analysis of the ventricles is achieved by obtaining a “stack” of contiguous cine short‐axis or axial images [49,62]. Quantification is then accomplished by tracing the endocardial and epicardial borders of both the LV and RV in both end‐diastole and end‐systole in order to obtain a volumetric ejection fraction [62]. Figure 47.2 and Video 47.1 depict the images and workflow required to obtain this type of information. More recently, 3D cine and ungated “real‐time” image acquisition techniques have been explored as alternatives to the more traditional 2D “stack” approach but have not yet been adopted in routine clinical practice. Biventricular analysis by CMR is possible in all cases of chamber enlargement, hypoplasia, and/or in complex forms of CHD such as single ventricle [61,63–65]. Unlike in the case of echo, RV mass can be obtained relatively easily by CMR and CCT, with good fidelity and reproducibility [65,66]. Assessment of ventricular diastolic function by CMR, although not as widely used as echo, is also available by CMR based on similar parameters as those obtained by echo [67–70]. In contrast, LV analysis by 2D echo relies on geometric assumptions, and the assessment of the RV is mainly done via surrogate measurements, such as fractional area change (FAC) and tricuspid valve annular plane systolic excursion (TAPSE), mostly validated in adults without CHD [71]. 3D echo quantification of RV size and function has been shown to be feasible in certain CHD patients with good CMR correlation, but requires specific software and expertise for accurate analysis [72–74]. However, 3D echo quantification of RV size and function is hampered by difficulties in acquiring full‐volume datasets, especially in adolescent and adult patients and those with RV dilation, which are the circumstances where accurate RV volume measurements are clinically most pertinent. Measurements of LV volume and function have long been utilized clinically with high fidelity in various patient populations [75–77]. Such measurements are helpful in diagnosis, follow‐up, and preoperative planning in patients with aortic valve disease, Shone complex, myocarditis, and the nonischemic cardiomyopathies (hypertrophic LV noncompaction, and dilated cardiomyopathy). LV mass is a clinical parameter associated with worse prognosis in patients with systemic hypertension, aortic stenosis, and hypertrophic cardiomyopathy, for example [78–80]. Measurements of RV volume and function have also proven crucial in the evaluation, follow‐up, preoperative planning, and postoperative surveillance in patients with repaired tetralogy of Fallot, sinus venosus defects, partially anomalous pulmonary venous connection, and arrhythmogenic cardiomyopathy, to mention just a few examples [14,81–83]. Determination of RV mass has been shown to have prognostic significance in patients with repaired tetralogy of Fallot [84]. Beyond its utility in the appraisal of cardiovascular anatomy and function, a technique known as phase contrast MRI yields valuable information on blood flow direction, velocity, and volume. Unlike echo Doppler, accuracy of flow data by CMR is not dependent on acoustic windows or angle of interrogation [85]. Figure 47.2 Short‐axis cine “stack” of steady‐state free precession CMR images at end‐diastole (a) and at end‐systole (b) with semiautomatically drawn left and right ventricular endocardial and epicardial contours. (c) Three reference planes that may be used to aid in proper contouring. (d, e) Phase contrast MRI flow analysis of the pulmonary valve. Flow versus time curve showing severe residual pulmonary regurgitation. (f) Numerical data are summarized in the table at the bottom right corner of the figure. By measuring flow in the aorta (Qs) and in the main pulmonary artery (Qp), shunt magnitude can be reliably quantified noninvasively through calculation of the pulmonary‐to‐systemic ratio [86]. Taken together with ventricular volume and function data, determination of shunt burden can be useful in quantifying the hemodynamic significance of shunt lesions such as atrial septal defects, sinus venosus defects, ventricular septal defects, anomalous pulmonary venous connections, patent ductus arteriosus, and aortopulmonary collaterals [87–89]. The distribution of pulmonary blood flow to each lung, for example in patients with repaired tetralogy of Fallot or reimplantation of the left pulmonary artery (e.g., left pulmonary artery sling repair), is also obtainable by this technique [90]. The information gleaned from calculation of the shunt fraction and the relative flow distribution to each lung can be additive to echo, particularly in cases where there is debate on whether or not transcatheter or surgical therapy is indicated, or in cases where echo is not conclusive [91]. Phase contrast MRI has also been employed in comprehensive assessment of cardiac valve function. The ability to visualize valve leaflet and apparatus, while also assessing the effect on valvular stenosis and/or regurgitation on cardiac chambers, can be helpful in deciding the optimal management strategy [50,52]. In the case of bicuspid aortic valve, CMR can aid in confirming the morphology and opening area of the valve as well as in providing valuable information on size and flow dynamics across the valve as well as in the ascending and descending aorta (Figure 47.3) [92–94]. The most common use of CMR for valvular assessment is in the case of residual pulmonary valve regurgitation in patients with repaired tetralogy of Fallot, which has become an indispensable follow‐up tool in this patient population [16,68,84]. Figure 47.4 depicts a case of repaired tetralogy of Fallot with severe residual pulmonary valve regurgitation resulting in severe RV enlargement and systolic dysfunction. Mitral and tricuspid valve functional assessment can be performed by CMR as well, but it is more limited due to challenges owing to significant through‐plane motion of the valve annuli and lack of standardized CMR definitions of severity [95]. However, in the absence of multivalve regurgitation or intracardiac shunt, the difference between RV and LV stroke volumes can be used to measure mitral or tricuspid valve regurgitation. Unlike the adult population, most coronary artery pathology in children centers on congenitally anomalous origins and the acquired condition Kawasaki disease. For various reasons, x‐ray angiography, CCT, and echo have been the preferred modalities of assessing coronary artery anatomy in children: x‐ray angiography and CCT for their superior spatial resolution, and echo for its portability and the lack of radiation exposure. More recently, however, CMR technology and sequences have improved to the extent that coronary artery imaging is not only feasible but potentially useful in children with abnormal coronary artery anatomy and in aneurysms due to the effects of Kawasaki disease [96,97]. Figure 47.3 Cardiovascular magnetic resonance evaluation of bicuspid aortic valve and coarctation of the aorta. (a) Flow analysis of the bicuspid aortic valve using phase contrast MRI is then applied to the same imaging position in order to quantify flow and velocity across the aortic valve. In this case, no stenosis or regurgitation were seen, as noted by a maximum velocity of 64 cm/s (top graph) and the lack of flow reversal below the baseline (bottom graph). (b) Contrast‐enhanced volume‐rendered MRA (posterior projection) of the associated coarctation of the aorta (arrow). Figure 47.4 (a–d) Cardiovascular magnetic resonance steady‐state free precession images prescribed in various imaging planes in a patient with repaired tetralogy of Fallot who has severe residual pulmonary valve regurgitation leading to right ventricular enlargement. (e) 3D volume rendering of the contrast‐enhanced MRA in the same patient. Shown are: (i) narrowing of the mid right ventricle–pulmonary artery conduit (asterisk in (c)); (ii) stenosis of the left branch pulmonary artery (white arrow in (d)); (iii) left aortic arch with aberrant right subclavian artery (yellow star in (e)); and (iv) a curious course of the innominate vein in between the right common carotid artery anteriorly and the left common carotid artery posteriorly (yellow arrow in (e)). LV, left ventricle; RV, right ventricle. Cardiovascular MRI has the unique ability to assess damage to the myocardium noninvasively and without the need for potentially risky cardiac biopsy [98]. Through techniques such as late gadolinium enhancement (LGE) imaging and parametric imaging (e.g., T1 and T2 mapping), information about myocardial edema, focal areas of fibrosis, and diffuse myocardial fibrosis can be evaluated and measured. Similar information is not as readily obtainable by echo or CCT. The LGE technique is most commonly applied in the assessment of adults with ischemic cardiomyopathy and valvular pathology [99–103]. However, applications to various conditions affecting adults and children with nonischemic cardiomyopathy, myocarditis, cardiac tumors, and various forms of CHD have also been recognized (Figure 47.5) [23,104–110]. Newer and more quantitative techniques of assessing myocardial fibrosis and remodeling have been developed and are being employed in addition to LGE imaging in the these conditions, including parametric mapping (T1, T2, and T2*) and calculation of the extracellular volume of myocardium for quantification of diffuse fibrosis [111]. Computed tomography (CT) imaging was first introduced in the early 1970s with the overall aim of overcoming the limitations of projectional x‐ray imaging such as scatter and tissue overlap [112]. However, its role in cardiac imaging was limited until the development of continuous gantry rotation, multidetector helical scanners, and ECG gating [113–115]. These technical improvements led to higher spatial and temporal resolutions, faster image acquisition, and the ability to resolve cardiac motion [116,117]. Over the past decade, with further advances such as the availability of dual‐source scanners, CCT has revolutionized coronary angiography, having a major impact on the care of patients with atherosclerotic heart disease [118–120]. Simultaneously, its role in children and adults with CHD continues to expand [29,121,122]. Figure 47.5 Late gadolinium enhancement imaging. (a, b) Delayed post‐contrast four‐chamber (horizontal long‐axis) and short‐axis views of a patient with hypertrophic cardiomyopathy caused by a LAMP‐2 mutation. Bright areas of myocardial fibrosis can be seen in the mid‐myocardial and endocardial layers throughout the left ventricular free wall, apex, and septum (arrows). (c) Short‐axis CMR image of a young adult with severe dilated cardiomyopathy secondary to Duchenne muscular dystrophy. Extensive epicardial distribution of delayed enhancement is noted, as is typical is this disease process (arrows). (d) Left ventricular outflow tract (vertical long‐axis) view of a patient with acute myocarditis. Shown is the typical patchy left ventricular mid‐myocardial and epicardial enhancement pattern (arrows). Multiple technical advances in CT technology over the past decade have made this imaging modality more accessible to children and adults with CHD. In particular, the availability of dual‐source scanners equipped with two x‐ray sources and two large detector arrays, and faster gantry rotation speeds, have substantially reduced scan times and improved temporal resolution [123,124]. The ability to scan the entire thorax within a single heartbeat eliminates respiratory and cardiac motion artifacts, obviating the need for sedation or anesthesia for a majority of indications. Simultaneously, other technical developments have resulted in marked reduction in the radiation dose [123]. The development of more powerful x‐ray tubes that can deliver a higher tube current (milliampere‐second, mAs) allows scanning at a lower tube potential (kilovoltage, kV). Since radiation dose increases linearly with higher mAs but decreases exponentially with lower peak kV (kVp), the overall dose is significantly reduced, while maintaining adequate signal‐to‐noise ratio. Coupled with ECG dose modulation, the development of newer model‐based iterative reconstruction, and noise reduction techniques, these advances have allowed CCT scans to be performed using effective radiation doses <1 millisieverts (mSv), which represents marked reduction compared with conventional single‐source 8–16 slice scanners [123,124]. In addition to lowering radiation dose, the use of a lower tube potential (70–80 kV) also improves image contrast, as the effective energy of the x‐ray beam in the range of maximum absorption moves closer to the k edge of iodine [125]. The ability to performed CCT without sedation and with a low exposure to ionizing radiation has favorably tilted the risk‐to‐benefit ratio for patients with CHD, leading to increased utilization. Modern cardiac‐capable CT scanners provide several scanning modes [126]. The traditional low‐pitch helical scan with retrospective ECG gating provides the best image quality but also delivers the highest radiation dose, since x‐rays are delivered throughout the cardiac cycle [123]. This scan mode is used sparingly when multiphase cine imaging is necessary, or for coronary angiography with rapid or irregular heart rates. Radiation dose using this technique can be reduced by using ECG dose modulation to limit peak radiation exposure to a small proportion of the cardiac cycle, using a lower radiation dose during the rest of the cardiac cycle. A prospective ECG‐triggered axial or “step‐and‐shoot” scan is a multistation axial scan that acquires data in multiple steps and stiches them together seamlessly into a single reconstructed stack of axial images. When used with a large detector array, a complete dataset can be obtained in two or three steps. Data acquisition starts after a R wave is detected but occurs only during a prespecified portion of the cardiac cycle, thereby minimizing radiation exposure compared with the traditional retrospectively gated scan. This scan mode is useful in coronary angiography in patients with a rapid heart rate, by allowing imaging during multiple cardiac phases, which can be helpful when various coronary segments have different resting periods. Many third‐generation dual‐source scanners provide a highly accelerated scan mode that can be used to acquire a complete 3D dataset within a single heartbeat and with the lowest radiation exposure (typically <1 mSv). This is implemented using either a prospectively triggered high‐pitch helical acquisition or a single prospectively triggered axial acquisition using a very large detector [126]. Although data on image quality are limited, several reports suggest that image quality is comparable to traditional scan techniques, despite a lower radiation exposure [123,127]. Available on modern scanning platforms from most vendors, these highly accelerated scan modes are becoming the standard of care for most pediatric and adult CHD applications, as they obviate the need for sedation in most cases, and deliver the lowest radiation dose, thereby making CCT more accessible. Modern third‐generation dual‐source CT scanners can provide a high‐resolution (voxel size 0.5 mm) 3D or 4D dataset of the entire chest that includes the cardiac chambers, the entire thoracic vasculature (including the coronary vessels), the airway, and the chest wall. The scans can usually be completed with a single peripheral injection of iodinated contrast, without need for sedation or breath‐holding, and completed within a few seconds (often in a fraction of a second). This rich 3D or 4D dataset can be interrogated and reconstructed to diagnose the most complex CHD. In addition, the relationship of vascular, airway, and chest wall structures to each other is readily visualized in 3D space, making this particularly suited for planning complex surgical and transcatheter interventions. A major drawback of CCT is the risk related to exposure to ionizing radiation. However, with recent technical advances, CCT using modern third‐generation dual‐source scanners can be performed with exposure to low doses of radiation. Because CHD patients often require repeat imaging, they should be scanned using these modern scanners whenever possible. Careful attention should be paid to scan parameters, in order to minimize radiation exposure. Secondly, although dual‐source scanners have an improved temporal resolution, it is still not comparable to the temporal resolution of echo or CMR images. As is the case with CMR, CCT is particularly suited for assessment of the extracardiac vasculature. This can be useful in assessment of aortic arch abnormalities such as coarctation and interrupted aortic arch (Figure 47.6) when echo evaluation is insufficient or incomplete (e.g., in older children or adults with poor echo windows) [128]. In patients with suspected vascular rings, the 3D depiction of the vascular components of the ring in relationship to the airway, esophagus, and chest wall can be useful in planning surgery. High‐resolution 3D assessment of the pulmonary vasculature with delineation of various sources of pulmonary blood flow can be useful in providing a roadmap for surgical and transcatheter treatment of patients with tetralogy of Fallot, pulmonary atresia, and major aortopulmonary collateral arteries, and other patients with pulmonary artery abnormalities (Figure 47.7) [129]. In patients with pulmonary venous abnormalities, CCT can be useful in planning treatment, as well as in the follow‐up of patients after intervention, particularly those with residual or recurrent pulmonary vein stenosis, in whom it can help in planning reintervention (Figure 47.8) [130]. In patients undergoing single ventricle palliation, CCT can be particularly useful in assessing the extracardiac vasculature. Some centers have reported the utility of CCT in evaluating patients prior to a bidirectional Glenn shunt, without the need for invasive angiography [131]. The role of coronary CT in the evaluation of adults with atherosclerotic coronary artery disease is well established [120,132]. Similarly, its role in the evaluation of patients with congenital coronary anomalies has been demonstrated [133,134]. In patients with congenital coronary anomalies, CCT offers advantages compared with other imaging modalities due to its higher resolution and 3D nature, making it the preferred modality for preoperative planning. In addition to making the diagnosis, it is possible to evaluate the anomaly in more detail by delineating the size and shape of the ostium, abnormal vessel angulation, length of the intramural or interarterial segment of the coronary, the change in caliber beyond the intramural segment, and vessel dominance (Figures 47.9 and 47.10). However, it should be noted that the prognostic significance of these anatomic features remains incompletely defined. In patients with Kawasaki disease, echo is useful in the diagnosis and follow‐up of patients with coronary artery aneurysms. However, in patients with giant coronary aneurysms, who are at risk for adverse coronary events, echo can be insufficient in delineation of the distal coronary artery anatomy. In these patients, CCT can be used instead of invasive angiography for serial assessment of complications such as coronary thrombosis, stenosis, and calcification (Figure 47.11) [135,136]. In patients with inherited hyperlipidemia, CCT can be useful in guiding treatment by providing serial assessment of plaque burden and coronary stenosis. Recently, the risk of coronary compression by epicardial pacing leads has been reported [137]. Cine CCT can be useful in assessing these patients for dynamic coronary compression (Figure 47.12). In patients with CHD in whom the coronary artery anatomy is relevant to the surgical repair (e.g., transposition of the great arteries prior to an arterial switch operation) and an inconclusive echo diagnosis, CCT can be useful in assessing coronary artery anatomy [123,138]. Finally, in patients with repaired CHD, CCT can be useful in evaluation for possible coronary artery compression or occlusion (Figure 47.13).
CHAPTER 47
The Role of Multimodality Cardiovascular Imaging
Introduction
Cardiovascular magnetic resonance imaging
Technical aspects
Strengths and limitations
Clinical indications
Cardiovascular anatomy
Valvular anatomy and function
Ventricular size and function
Hemodynamic data
Coronary artery assessment
Tissue characterization
Cardiac computed tomography
Technical aspects
Scan modes
Strengths and limitations
Clinical indications
Extracardiac vascular abnormalities
Coronary artery assessment
Stay updated, free articles. Join our Telegram channel
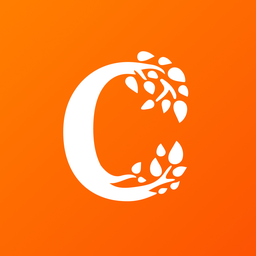
Full access? Get Clinical Tree
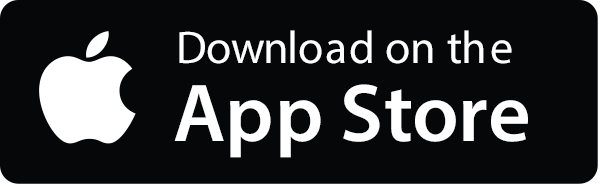
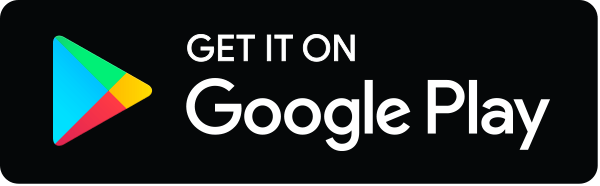