44 Luke A.Wittenburg and Daniel L. Gustafson The current era of chemical anticancer therapy took hold in the mid-20th century, yet its roots can be traced back 50 centuries to ancient descriptions of neoplastic diseases and the use of medicinal herbs and botanicals in the treatment of these conditions (Papac, 2001; DeVita and Chu, 2008; Morrison, 2010). Nitrogen mustard, the first modern pharmacological chemotherapeutic agent, was developed in the 1940s and additional drugs derived from nitrogen mustard followed shortly after (DeVita and Chu, 2008). The first reported case of treatment of cancer in veterinary medicine occurred in 1946 and involved the use of urethane to treat a dog with lymphoma and lymphocytic leukemia (Innes et al., 1946). The first facility devoted entirely to the treatment of cancer in dogs, the Canine Cancer Clinic, was founded in 1950 and within 10 years had published a review of chemotherapy treatment of spontaneous cancers in canines using numerous agents ranging from nitrogen mustards to cortisone (McCoy, 1958; Morrison, 2010). It was suggested as early as 1962 that the treatment of animal cancer patients had become an obligation of the veterinarian to his or her community (Morrison, 2010). With the advent of animal models of cancer that were predictive for antineoplastic drug activity, drug screening programs were initiated in the 1950s that ultimately resulted in the discovery and development of many of the agents in clinical use today. Since that time, significant advances have been made in the understanding of the biology of cancer and response of specific tumor types to various drugs. While direct translation of discoveries made in human oncology has been hampered, in part by species differences in drug pharmacokinetics, expense, and lack of widespread availability of adequate supportive care in general practices, there has been a significant improvement in the quality, and in many cases quantity, of life for pet cancer patients through improved client education and rational decisions in the selection of appropriate patients while matching therapeutic regimen with therapeutic goal. Chemotherapy is defined as the application of drugs in an attempt to kill or inhibit the growth of viruses or foreign cells such as bacteria or fungi in the body. Although originating from the individual, cancer cells are viewed as “foreign” in this sense. Recent advances in understanding the biology of cancer and cellular signaling networks important in cancer cell survival and proliferation have led to the development of targeted therapies, usually small molecules or antibodies directed at specific cellular receptors or proteins. In contrast to these therapies directed at tumor cells, cancer immunotherapy can have an indirect action through potentiation or reactivation of antitumor immune responses that tumors often suppress as a means of escaping immune recognition. Regardless of the type of therapy employed, the treatment regimen chosen must be appropriate for the therapeutic goal for the individual patient. A cure is defined as the complete eradication of all tumor cells within the body; in most cases this is not achievable and palliation, the reduction of pain and improvement of function to enhance quality of life in the absence of a possibility of cure, has become increasingly recognized as an acceptable therapeutic goal. The ratio of the toxic dose to the therapeutic dose of a drug is termed the therapeutic index. This is often extremely small in cancer chemotherapy as the dose that causes a desired effect, such as reduction in tumor size, is often anticipated to cause some degree of toxicity. Furthermore, the vast majority of chemotherapy drugs are administered at the maximum tolerated dose (MTD) which is defined as the highest dose of a drug that can be given without unacceptable or irreversible adverse events and is generally empirically derived in a small population of animals. Toxicities following drug administration in veterinary patients is scored based on guidelines set forth in the Veterinary Co-operative Oncology Group Common Terminology Criteria for Adverse Events (VCOG-CTCAE) (VCOG, 2011) and are used to guide dose adjustments for subsequent treatments. More recently, the advent of targeted therapy has given rise to the concept of a biologically effective dose (BED), defined as the dose leading to some measurable desired response at the proposed target or a surrogate biomarker. Dosing of chemotherapy drugs in humans and animals is generally based on body surface area (mg/m2) instead of patient weight (mg/kg). This was originally instituted as a means of normalizing the maximum tolerated dose of drug between species and was based on the idea that physiological processes that influence drug activity in the body correlate better with body surface area than weight. The exception to body surface area based dosing in veterinary medicine is in very small patients where this dosing results in a relatively higher dose and often weight based dosing may be chosen for animals weighing less than 10 kg. The formula for converting weight to body surface area for dogs and cats is: where surface area is given in square meters and K is a species constant with a value of 10.0 and 10.1 for cats and dogs, respectively. Numerous tables exist for conversion of weight to surface area. The decision to use a certain chemotherapeutic drug or combination of drugs, with or without additional therapeutic modalities such as surgery and radiation therapy, as well as the assessment of response, is influenced by the therapeutic intent for each individual patient. Therapeutic decisions are influenced by patient factors such as species, breed, age, and health status, neoplasm factors such as histological type, stage and/or grade of tumor, facility factors such as availability of quality follow-up care and available treatment modalities, and pet owner factors such as time commitment, tolerance for adverse effects, and cost of therapy. Adjuvant therapy, where chemotherapy is utilized following control of the primary tumor by means such as surgery or radiation, aims to limit the spread of micrometastatic disease and is a common application of chemotherapy in veterinary medicine. Neoadjuvant or primary therapy is the use of chemotherapeutic drugs prior to surgery or radiation as a means of limiting spread of metastatic disease as well as reducing primary tumor burden. In reference to hematopoetic or lymphoid tumors, induction therapy is a term referring to the initial utilization of chemotherapy drugs to induce a remission. Following induction of remission, maintenance therapy involves the use of chemotherapy with the goal of maintaining a stable remission. When a tumor fails to respond to chemotherapy or a patient comes out of remission, rescue or salvage therapy is often employed with the goal of achieving a second remission. A basic understanding of some of the aspects of the biological behavior of cancer is important for understanding the therapeutic approaches and the limitations of these approaches due to the striking diversity of neoplastic diseases. The transformation of normal cells to neoplastic cells can be viewed as a multistep process by which normal cells acquire successive capabilities that allow for tumor growth and subsequent spread throughout the body. These capabilities have been described as “hallmarks” of cancer and are traits that differentiate cancer cells from normal cells in the body, the most fundamental being the ability to sustain signaling that allows the cell to continuously proliferate (Hanahan and Weinberg, 2011). In addition to sustaining positive proliferative signaling, cancer cells must also bypass the programs that are designed to inhibit proliferation and maintain homeostasis of cell numbers and normal tissue architecture through control of entry into the cell growth and division cycle (Hanahan and Weinberg, 2011). While the biochemical processes of cell proliferation and passage through the cell cycle are the same between cancer and normal cells, it is the rate and timing of the processes that provide a qualitative difference and results in the utilization of drugs that target rapidly dividing cells as a therapeutic option. Because many of the drugs are most active against cells that are actively dividing, and some chemotherapy agents are more active in specific phases of the cell cycle, an understanding of the activities that occur during each phase of the cell cycle is important. The cell cycle is generally divided into four phases: G1, S, G2, and M, with cells that are at rest or not actively dividing termed G0. The G1 phase is a period of cell growth, active gene transcription, and protein synthesis and is required for building up the “biomass” required for cell division. This phase is followed by the S phase, a period of DNA replication and chromosome duplication in preparation for mitosis. During the G2 phase the cell continues to grow in size and in M phase the cells undergo active mitosis. Prior to the start of the S and M phases of the cycle are two important checkpoint mechanisms, which cells utilize to ensure that the cell is prepared to carry out chromosome duplication and mitosis, and these checkpoints are often bypassed in cancer cells allowing for uncontrolled growth. Figure 44.1 depicts the cell cycle specificity of the major classes of drugs that are used in veterinary medicine. Other hallmarks of cancer have also provided some potential therapeutic targets. While sustaining positive proliferative signaling and evading growth-suppressive signaling are important for cancer on the cellular level, in order to grow into a clinically relevant mass a tumor must induce angiogenesis, or the formation of new vasculature, in order to provide for the increasing demand for nutritional support (Folkman, 2002). Targeting tumor vasculature has been proposed as a way to control tumors by effectively starving them to prevent further growth (Kerbel and Folkman, 2002). The term metronomic chemotherapy refers to the use of low-dose, continuous administration of conventional cytotoxic drugs without breaks in treatment and has been utilized successfully in human and veterinary oncology as a means to delay tumor recurrence or slow growth (Elmslie et al., 2008; Burton et al., 2011; Romiti et al., 2013; Schrempp et al., 2013). Figure 44.1 Cellular targets of the common classes of chemotherapeutic agents used in veterinary oncology and their sites of activity. While uncontrolled cellular growth and passage through the cell cycle are a hallmark of cancer cells, the growth rate of a tumor in the clinical setting does not necessarily directly correlate with the rate at which cells are traversing the cell cycle. Early studies into the kinetics of cell proliferation in experimental tumors demonstrated that tumor growth rate is rapid initially but then slows as the fraction of cells entering the cell cycle reduces and cell death increases, potentially due to reduced availability of nutrient supply in bulky tumors; in each examined stage of tumor growth the length of the cell cycle itself remained unchanged (Frindel et al., 1967). Clinically, many tumors in veterinary medicine are diagnosed when they are relatively advanced and thus there may be only a small fraction of cells that are actively undergoing cell division, which has implications for effectiveness of therapy when utilizing drugs that have their maximal effect on actively dividing cells. In the 1960s, studies in mice utilizing leukemia cell lines demonstrated that a single cell was capable of generating a tumor and that a cure could only be attained by eradication of all tumor cells (Skipper et al., 1964). Furthermore, it was determined that any effective dose of chemotherapy killed cells by log kill kinetics, which led to the fractional kill hypothesis. This states that with each dose of chemotherapy a constant fraction of cells is killed, as opposed to a constant number of cells, regardless of the size of the original tumor and that successful therapy is then dependent upon the number of cells present at the beginning of treatment. Thus, in theory, with the application of multiple doses of chemotherapy it should be possible to attain a cure. In practice this is not always the case as the fraction of cells killed with many of the available drugs is small, resistance to therapy often occurs, and toxicities prevent rapid successive administration of drugs. However, these concepts illustrate the importance of early detection in a successful treatment outcome as smaller tumors are likely to have a larger dividing fraction and the fractional kill hypothesis implies that a cure could be achieved earlier in the course of treatment for smaller tumors with fewer cells present. Increased understanding of cancer cell biology has led to the evaluation of a number of novel targets for therapy. The targets for traditional cytotoxic chemotherapy include DNA (through targeting of nucleotide bases, enzymes involved in DNA synthesis, and DNA damage repair), microtubules, and growth factor receptors. More recently discovered targets include products of over-expressed oncogenes, antiapoptotic proteins, cell-cycle regulators, proteasomal machinery, cell surface antigens, and enzymes involved in epigenetic modulation of DNA. While cancer in veterinary patients may share many of the same putative targets as human cancers, translation of newer “targeted” therapies into veterinary medicine has lagged behind development in human oncology, with a couple of notable exceptions. This chapter will focus on the drugs that are currently in primary use for veterinary oncology. Individual cell sensitivity to a drug is dependent upon a number of factors, such as absorption, distribution, and metabolism, entry into the cell or affinity of interaction with a cell-surface target, the type of lethal or sublethal damage induced, and the cellular response to this damage. Altered tumor vasculature and differences in intratumoral pressure or drug binding can affect drug uptake in a negative or positive way. Some drugs can enter the cell through passive diffusion due to the lipophilic nature while others may utilize active transport via an array of carriers such as amino acid transporters (melphalan), nucleoside transporters (gemcitabine), or reduced folate carriers (methotrexate)(Goldman et al., 1968; Mackey et al., 1998; Yanagida et al., 2001). The importance of active transport mechanisms for entry into the cell is that reduced entry, and thus diminished efficacy, can occur with administration of other substrates or analogs that block drug transport. Many cytotoxic drugs exhibit greater toxicity in rapidly dividing cells and are termed cycle specific. There are a few drugs that have similar effects on proliferating and nonproliferating cells, including the nitrosureas (cyclohexylchloroethylnitrosourea, CCNU, and bischloroethylnitrosourea, BCNU)(Twentyman and Bleehen, 1975). Because they do not require cells to be proliferating to exert lethal effects these drugs are termed cycle nonspecific. Other cytotoxic drugs may demonstrate variations in lethal toxicity depending on the phase of the cell cycle and are termed phase specific. Vinca alkaloids and taxanes are examples of drugs that exert lethal damage exclusively in cells undergoing mitosis as they target the dynamics of microtubule formation. For drugs that have a very direct and specific interaction with a target molecule, the cellular levels of the target can affect the intrinsic sensitivity of the cell. For example, doxorubicin and other anthracyclines interact with topoisomeraseII-α (TopoII) and reduced levels of this target have been identified in populations of doxorubicin-resistant cells (Withoff et al., 1996). Furthermore, amplification of the gene encoding TopoII, leading to increased target expression, has been identified as a potential marker of enhanced sensitivity and improved outcome with anthracycline-based chemotherapy in human breast cancer patients (Park et al., 2003). For drugs that directly target DNA, the sensitivity of a cancer cell is dependent upon the balance between formation of DNA lesions and repair of the lesions. Alkylating agents such as cyclophosphamide exert their toxic effect by inducing DNA strand breaks following the formation of O’6-methylguanine; the enzyme O’6-methylguanine-DNA methyltransferase (MGMT) is a conserved protein that is capable of directly reversing this lesion and levels of MGMT have been proposed as a predictor of tumor sensitivity to alkylating agents in humans (Hansen et al., 2007; Xu et al., 2014). Often, the final effector of cancer cell death following exposure to cytotoxic drugs is the activation of apoptosis, or programmed cell death. The activation of apoptosis in a cell following a toxic insult is dependent upon the balance of pro- versus antiapoptotic signaling, and alterations that shift the balance in the direction of antiapoptosis, as has been demonstrated in human and canine lymphoma with elevated levels of the antiapoptotic protein survivin, have a negative impact on chemosensitivity (Ambrosini et al., 1997; Rebhun et al., 2008). Thus, the proximity of a cancer cell to the “apoptotic threshold”, determined by the interplay between the pro- and antiapoptotic signals, can be a key determinant of therapeutic responsiveness. Currently, there are efforts to utilize the in vitro chemotherapeutic sensitivity of large panels of cell lines, such as the NCI 60, along with gene expression data from these cells, to begin to build models that might predict sensitivity of other cell and tumor types based on similarities in gene expression signatures, and validation of these models may allow the future administration of cytotoxic agents in a more personalized manner (Scherf et al., 2000; Lee et al., 2007). Resistance to chemotherapy is the major mechanism of therapeutic failure in cancer drug therapy. Many of the hallmarks of cancer and the genetic instability of cancer cells provide mechanisms whereby cells can tolerate and sustain the stresses induced by cytotoxic drugs. Resistance can be either intrinsic to the cell or acquired through the selection and subsequent expansion of resistant clones as sensitive cells are killed. Intrinsic resistance mechanisms are those that are constitutively expressed in the tumor tissue from the outset of therapy as they are generally derived from the normal tissue from which the tumor developed. As a component of their barrier function, epithelial tissues generally express numerous transport and detoxifying enzymes for xenobiotics, and carcinomas derived from these tissues also often express increased levels of these enzymes leading to a more resistant phenotype. Conversely, tumors derived from normal tissues with lower levels of defense mechanisms, such as hematopoietic or lymphoid tissues, are relatively more chemosensitive than epithelial tumors. As mentioned in the previous section, altered drug uptake can influence the sensitivity or resistance level of a cancer cell to drugs such as melphalan, methotrexate, or nucleoside analogs such as gemcitabine. Some cytotoxic agents are administered as prodrugs and require metabolic or catabolic activation to an active form to result in cell death. Reduced activation has been identified as a potential mechanism of acquired resistance for the nucleoside analog group of drugs and has been suggested as a mechanism for resistance to the alkylating agents cyclophosphamide and ifosfamide (Spears, 1995; Zhang et al., 2005). When administered as single agents, that is not as part of a combination therapy protocol, resistance to some drugs manifests as a direct modification of the drug target. Resistance to antimetabolite drugs such as methotrexate and 5-fluorouracil has been found to involve either direct mutation of the target enzyme that reduces binding affinity or mutation that results in decreased levels of the target directly (Gonen and Assaraf, 2012). Similarly, target-based mechanisms of resistance have been identified for the microtubule binding drugs such as taxanes, vincristine, and vinblastine. As these drugs disrupt normal tubulin dynamics through their interaction with β-tubulin, increased expression of different cellular isoforms of β-tubulin with lower binding affinity has been identified, as has mutation in the target β-tubulin that also results in reduced binding affinity (Monzo et al., 1999; Rebucci and Michiels, 2013). For the group of drugs that target DNA replication through interactions with topoisomerase I or II, mutations in the gene encoding the topoisomerase enzyme have been identified, and cells with reduced levels of topoisomerase II demonstrate resistance to doxorubicin (Pilati et al., 2012; Tomicic and Kaina, 2013). In addition to qualitative and quantitative alterations in the interaction of cytotoxic drugs with their targets, resistance can also develop through increased metabolism or detoxification of a drug by cancer cells. Many of the currently used cytotoxic agents interact with DNA through electrophilic interactions, making them sensitive to thiol-mediated inactivation by conjugation with nucleophiles such as glutathione (Tew, 1994). Induction of the enzyme responsible for conjugation of glutathione to cytotoxic drugs, glutathione S-transferase (GST), has been demonstrated in leukemia cells that have acquired resistance to alkylating agents and there is evidence that this may also be a mechanism through which tumors can acquire resistance to platinum agents such as cisplatin (Schisselbauer et al., 1990; Tew, 1994). In addition to detoxification through conjugation, metabolic inactivation of drugs is implicated as a mechanism of resistance in some instances. Acquired resistance to the nucleoside analog 5-fluorouracil can be associated with elevated cellular levels of dihydropyrimidine dehydrogenase (DPD), the main enzyme responsible for metabolism of fluorouracil to an inactive compound, and DPD activity in tumor tissue has been utilized as a potential marker in determining whether a particular tumor is likely to demonstrate resistance to therapy (Baba et al., 2003; Rebucci and Michiels, 2013). As the cellular target of many cytotoxic agents is ultimately the DNA, alterations in the response to DNA damage will determine the fate of the cell exposed to these drugs. In normal cells, the DNA damage response is intimately related to cell cycle progression, and detection of damaged DNA generally leads to cell cycle arrest to allow for repair or, if damage cannot be repaired, apoptosis. Resistance to cytotoxic agents can occur through up-regulation of DNA repair processes that abrogate the effects of DNA damaging agents or through down-regulation of damage recognition, both of which act to prevent induction of apoptosis. Altered activity of repair processes specific to double-strand breaks, single-strand breaks, single-nucleotide excision, and base mismatch repair have all been found to play some role in the acquired resistance to a diverse group of cytotoxic agents, including the anthracyclines, camptothecins, alkylators, and platinum agents (Parker et al., 1991; Fink et al., 1998; Pommier et al., 1999; Martin et al., 2008; Helleday, 2010). Some general mechanisms of acquired resistance to cytotoxic agents are listed in Table 44.1. Table 44.1 General mechanisms of acquired resistance leading to therapeutic failure for some cytotoxic agents One very concerning mechanism of acquired resistance results in a phenotype in which cancer cells become resistant to a variety of structurally and mechanistically diverse drugs. While alterations in DNA repair processes or detoxification via glutathione conjugation can certainly lead to resistance to more than one drug, the classic mechanism resulting in the multiple drug resistance (MDR) phenotype involves the increased removal of drugs from the cell. This is more commonly encountered following therapy with multiple drug protocols where resistance does not appear to occur simply through a combination of target-directed alterations that confer resistance to the individual components of the protocol, but through a more global phenotypic change toward chemoresistance (Pritchard et al., 2012). The MDR phenotype generally refers to the increased expression of energy-dependent integral membrane transport proteins that function to remove drugs from the cell following exposure to chemotherapy (Table 44.2). The major family of proteins that has been implicated is the ATP-binding cassette transporter (ABC) family, comprising approximately 48 proteins, of which 15 are known to have chemotherapeutic drugs as substrates (Fletcher et al., 2010). The most well studied of these proteins is P-glycoprotein, a product of the MDR1 gene (ABCB1), which has been implicated in resistance to a number of lipophilic cytotoxic drugs including the vinca alkaloids, anthracyclines, epipodophyllotoxins, taxanes, actinomycin D, topotecan, methotrexate, and mitomycin C (Fletcher et al., 2010). Aside from being expressed in some tumor tissues, ABCB1 is also expressed in normal cells that line tubules and ducts, serving a role that allows for removal or prevention of uptake of xenobiotics into these cells. Tissues such as kidneys and adrenals, liver and pancreas, endothelial cells (particularly those of the blood–brain barrier), small intestine and colon, and testes all express varying levels of ABCB1. Therapeutic implications of the expression of ABCB1 in nontumor tissues include reduced absorption of orally administered substrates requiring intravenous administration to achieve effective levels and hastening the elimination of substrates from the kidney and liver. Importantly, a loss of this normal barrier function has been demonstrated to result in increased toxicity from substrate drugs such as that seen in Collie dogs and related breeds following exposure to ivermectin or loperamide manifesting as neurotoxicity (Mealey et al., 2001; Sartor et al., 2004). In addition, increased toxicity of substrate chemotherapeutic drugs has been identified in the same population even at reduced doses (Mealey et al., 2003), and pharmacokinetic modeling suggests that this population of dogs may not be able to safely receive substrate drugs such as doxorubicin at doses that are tolerable yet retain efficacy (Gustafson and Thamm, 2010). A more complete review of the therapeutic implications of the MDR1 gene has been published previously (Mealey, 2004) and is discussed in Chapter 50. Table 44.2 Examples of ABC transporters implicated in MDR and cytotoxic agents identified as substrates a Identified as substrates for mutated forms of the protein but not wild-type (Meyer et al., 2011). ABC, ATP-binding cassette; BCRP, breast cancer resistance protein; MDR, multiple drug resistance; MRP, multidrug resistance-associated protein. The other ABC transporters that have been studied the most extensively with regard to a role in acquired resistance to chemotherapy are the BCRP (ABCG2), MRP1 (ABCC1), and MRP2 (ABCC2) proteins (Wu et al., 2011). The importance of these transporters has been less extensively studied and their importance in the MDR phenotype in veterinary cancer therapy is less well defined. Another transporter that does not belong to the classical ABC family of membrane proteins has been implicated in resistance through redistribution of drug within the cell resulting in accumulation away from the target. The major vault protein (MVP/LRP) has been found to cause sequestration of anthracycline drugs within cytoplasmic organelles preventing their interaction with topoisomerase enzymes (Kickhoefer et al., 1998). Clinical studies from human oncology have attempted to address MDR in cancer through administration of various inhibitors of ABC transporters, most often ABCB1; however, results have been disappointing and explanations for these results include the functional redundancy of some transporters in addition to the fact that often more than one is up-regulated, as well as an increase in systemic toxicity most likely due to altered pharmacokinetics with regard to reduced clearance mechanisms (Fletcher et al., 2010). Veterinary cancer patients may frequently be treated with a combination of chemotherapy drugs or with multimodal therapy, which combines chemotherapy with other modalities such as surgery or radiation therapy. Combination therapy mainly provides a mechanism whereby tumors are exposed to increased dosages of drug which subsequently provides for increased fractional cell kill. The term summation dose intensity (SDI) is used to express the relationship between the number of agents in a combination and the overall dose, and can be increased through either adding more agents to the combination or increasing the dose of individual agents. For a combination chemotherapy protocol to provide a therapeutic advantage, the increased toxicity to cancer cells must be greater than any increase in damage to critical normal tissues. The implementation of combination therapy in clinical practice is based in the early studies of leukemia models in mice, which were then translated into treatment of childhood leukemia and resulted in the first major improvements in both the rate and duration of remission and resulted in the first responses that were compatible with a cure (Law, 1952). One of the driving factors in development of combination therapies relates to heterogeneity within tumors with regard to cytokinetics; with a tumor mass containing both cycling and noncycling cells, the use of cycle-specific agents to kill mitotically active tumor cells and cycle nonspecific agents to kill noncycling tumor cells was empirically employed. Furthermore, the reduction of tumor mass using cycle nonspecific drugs may actually increase the growth fraction of a tumor through various mechanisms and increase the sensitivity to cycle-specific drugs (Frei and Antman, 2000). The rational development of combination protocols is based on a number of factors, such as independent or nonoverlapping toxicities, allowing for use of each drug at a full dosage, and each component of the combination should demonstrate some degree of efficacy when used as a single agent. In addition, use of drugs with nonoverlapping mechanisms of action and resistance may provide an improved therapeutic benefit when used in combination. Consideration should be given to the schedule of drug administration (i.e., the order in which drugs are given and timing between drugs) in combination therapy as the effects of the schedule can be quite substantial, although it is less well understood than the effects of dose. The best studied example of the superiority of combination therapy in veterinary oncology is in the treatment of lymphoma in dogs, in which combination protocols provide an improved response rate and duration of first remission when compared to numerous single-agent protocols (Chun, 2009). With regard to cytotoxic chemotherapy protocols, pharmacodynamic considerations relate to standard measures of response and in veterinary medicine have been adopted from the Response Evaluation Criteria in Solid Tumors (RECIST) guidelines used in human oncology (Eisenhauer et al., 2009). Terms that are important in the clinical evaluation of cancer therapeutics involve measurements of tumor shrinkage and tumor burden versus disease progression: Progression-free interval/progression-free survival (PFI/ PFS): the amount of time elapsed without evidence of either progressive tumor growth (PFI) or survival without progressive growth of tumor measured from the time of initiation of therapy (PFS). With the introduction of newer targeted agents in veterinary medicine, pharmacodynamic responses can also be evaluated by detection of direct inhibition of the molecular target. Studies of the c-KIT inhibitor toceranib have demonstrated that, when used at clinically effective doses, reductions in the amount of activated KIT protein can be detected and these changes correlate with the plasma concentrations of drug (Pryer et al., 2003). Identification of these types of biomarkers of drug activity and their correlation with plasma concentrations may become more important as the optimal dosing of these types of targeted therapies may not be based on MTD but on the biologically effective dose required to inhibit a molecular target. Aside from the determinants of sensitivity and mechanisms of resistance described above, failure of a given therapeutic protocol may also be a result of treatment discontinuation due to unacceptable toxicity. Phase I studies specific to companion animals for a number of cytotoxic agents have been performed and the MTD defined from these studies is generally the suggested starting dose. However, there is substantial inter- and intraindividual variability with regard to efficacy and toxicity following equivalent doses in patients. For example, in human patients following standard doses of some drugs the variability in drug exposure can range between two and tenfold (Masson and Zamboni, 1997). The pharmacokinetic parameters of many anticancer agents are associated with outcome in terms of response and toxicity, and the high degree of variability makes for inaccurate predictions of response based solely on dose administered. A complete review of the reasons for pharmacokinetic variability in cancer is beyond the scope of this chapter, but patient variability with regard to PK and patient physiology and altered physiological states has been reviewed elsewhere (Undevia et al., 2005; Martinez and Modric, 2010; Modric and Martinez, 2011). With a few drug-specific exceptions, cytotoxic chemotherapy drugs generally produce a characteristic set of toxicities to various tissues that are often related to the tissue growth rate; given appropriate time most if not all of these tissues will recover. In fact, current convention of scheduling in combination protocols is determined by the length of time it takes for normal tissues to recover following cytotoxic insult. In addition, it is the development of improved supportive care procedures that often allows for more dose-intense treatment protocols. Thus, the selective therapeutic advantage of “nontargeted” or general cytotoxic agents lies in the fact there is often more rapid and complete repair of damage in normal cells than cancer cells. Toxicities that develop following chemotherapy administration can be placed into three categories: (i) acute toxicity that generally occurs during treatment or very shortly thereafter, (ii) acute-delayed toxicity which generally occurs within 2–14 days following administration, and (iii) chronic or cumulative toxicity that can develop anywhere from weeks to years following administration. Acute toxicity can manifest as hypersensitivity reactions that occur during infusion and result from histamine release caused by either the active drug (L-asparaginase, doxorubicin) or from vehicle-mediated mast cell degranulation (paclitaxel and etoposide). Treatment of this hypersensitivity often involves stopping administration of the drug and general symptomatic therapy for allergic reactions and anaphylaxis such as antihistamines and/or steroids. Routine pretreatment with antihistamines in patients with known hypersensitivity to a given drug may also be effective in preventing acute reactions. Acute reactions may also present as nausea and vomiting that can be intrinsic to the drug (cisplatin) or a function of a rapid rate of infusion (doxorubicin). Pretreatment with antiemetic drugs and increasing length of infusion can be effective means to prevent acute vomiting. Examples of potential cumulative/chronic toxicities include dilated cardiomyopathy in dogs when cumulative doxorubicin doses exceed 180 to 240 mg/m2, hepatic dysfunction following administration of CCNU, and renal failure following cisplatin use in dogs or doxorubicin in cats. It is important to understand that these toxicities could potentially develop after only a single administration and thus do not always present following chronic administration or follow a protracted course of appearance. Careful evaluation of clinical and laboratory indices prior to administration and careful monitoring during treatment, as well as prudent choice of drug, are critical in preventing or reducing severity of these toxicities. Secondary malignancies are reported for some of the cytotoxic agents due to their mitogenic potential following DNA damage. This is not commonly encountered in veterinary medicine as the length of time between drug administration and the development of a secondary malignancy is often longer than most pets survive. Here again there are differences in the ability to cause secondary malignancies between drugs with the alkylating agents being the most mutagenic and vinca alkaloids among the least mutagenic. The delayed acute effects are most commonly encountered following cytotoxic chemotherapy and some degree of these effects is generally expected. Tissues that seem to be particularly affected following the majority of cytotoxic agents include bone marrow, gastrointestinal mucosa, gonads, and hair follicles (tissues in which cells require constant renewal due to a short lifespan). Lymphocytes and bone marrow cells appear particularly sensitive to cytotoxic agents and neutropenia is often the dose-limiting toxicity (DLT). The low point in neutrophil count following chemotherapy is referred to as the nadir and is generally thought to occur 7–14 days following treatment with most drugs. Severe neutropenia can adversely affect treatment as it can result in dose reductions, treatment delays, and sepsis. Careful monitoring of leukocyte count is critical for appropriate management of neutropenia with regard to appropriate supportive care and future dose determination. Consideration of drugs used in combination protocols must account for the propensity of specific drugs to result in neutropenia as some drugs are more prone to result in significant myelosuppression (cyclophosphamide, carboplatin, doxorubicin, CCNU, vinblastine) while others may cause minimal neutropenia (bleomycin, vincristine in dogs, L-asparaginase). In cases of severe myelosuppression the administration of a recombinant granulocyte-colony stimulating factor (G-CSF) can increase circulating neutrophils by stimulating progenitor cell proliferation and differentiation (Fernandez-Varon and Villamayor, 2007). In addition to the anticipated myelosuppression following cytotoxic chemotherapy, gastrointestinal toxicity is another common sequela and presents as nausea/vomiting and diarrhea. Gastrointestinal toxicity in dogs and cats is often not as predictable as it is in humans given cytotoxic chemotherapy, and in many cases this is self-limiting and can be managed with general supportive care. In particularly sensitive dogs and cats GI toxicity may be severe and may require intervention such as antiemetics and/or antimicrobials or result in dose reduction. Metronidazole and tylosin are common choices of drugs for chemotherapy-induced diarrhea; however, if gastrointestinal symptoms accompany a febrile neutropenia then antibiotic choice will need to consider bacteria likely to result in sepsis. Unique toxicities specific to some drugs are discussed in the sections on each drug. Several of the commonly used cytotoxic agents can cause phlebitis and have the potential to create necrosis and tissue sloughing if extravasation occurs. Thus, it is critical that venipuncture and catheter placement are done appropriately and sedation or treatment delay may be necessary if this cannot be performed. Extravasation injury may occur up to 2–4 weeks following treatment and can continue for up to 4 months. Treatment will depend on the agent used; doxorubicin which causes the most severe extravasation injury should be treated by aspiration of any fluid present through the catheter or needle followed by ice-pack treatment three to four times per day. Extravasation of vinca alkaloids should also be addressed by aspiration of any liquid still present in the local area followed by heat therapy three to four times per day. In cases of doxorubicin and vinca alkaloid extravasation, intravenous dexrazoxane or local hyaluronidase administration, respectively, may reduce the subcutaneous toxicity (Schulmeister, 2008). Specific treatment guidelines for common chemotherapy induced toxicities encountered in veterinary oncology have been reviewed in more detail by others (Vail, 2009; Gustafson and Page, 2013). Hair loss is not a common side effect across all breeds of dogs or cats following chemotherapy but alopecia may be anticipated in breeds of dogs with continuously growing haircoats such as poodles and mixed-breed dogs of poodle lineage, as well as some terriers and Old English Sheepdogs. Cats may lose whiskers following chemotherapy. Regrowth may begin within 1 to 2 months following therapy; however, pet owners should be informed that hair regrowth may occur with slightly different color or texture than the original hair. Communication with pet owners regarding potential side effects prior to selection of a chemotherapeutic protocol is of utmost importance in reducing the distress that is experienced when a pet develops toxicity. In addition to toxicity experienced by the pet, exposure of the therapist should be considered when administering chemotherapy. Special consideration must be given for proper storage, preparation, administration, and disposal of cytotoxic drugs. The preparation of these drugs should be performed under strict guidelines that involve the use of appropriate personal protective equipment including gloves, masks, and chemical fume hoods. Specific guidelines for cytotoxic drugs are available from the Occupational Safety and Health Administration (OSHA). The potential for exposure of pet owners to chemotherapy residues following treatment generally involve exposure to urine or feces and variable levels of drug may be present in urine depending upon the particular drug and time since administration (Knobloch et al., 2010). Owners should be informed of the potential risk and importance of careful avoidance, collection, and disposal of urine and feces. Young children, pregnant women, and immunocompromised individuals should be instructed to avoid pet wastes for a defined period of time following chemotherapy administration. The alkylating agents were the earliest nonhormonal drugs utilized in the treatment of cancer and comprise a chemically diverse group of compounds. The mechanism of action of the alkylating agents is to interfere with the accessibility of DNA base pairs during replication through the covalent bonding of alkyl groups (e.g., –CH2Cl) to electron-rich nucleophilic groups such as phosphate or hydroxyl moieties. Although a large number of cellular macromolecules contain nucleophilic groups, which are potential sites of alkylation, it is the alkylation of DNA bases that is the major cause of cell death following treatment with alkylating agents. The N-7 and O’6 position of guanine are often a site of alkylation and the net effect can be the loss of guanine from the DNA strand with a resultant strand break. Because their action in causing DNA strand breaks resembles that of radiation, the alkylating agents are frequently referred to as radiomimetics. These drugs can contain one or two reactive groups and are classified as mono- and bifunctional, respectively. The bifunctional alkylating agents are capable of producing inter- or intrastrand DNA cross-links as their major mechanism of cytotoxicity. As a group, these drugs have limited cell cycle specificity and sensitivity of cells is relative to the area under the concentration time curve. In general, the alkylating agents are most useful in tumors of lymphoreticular origin and are less commonly used in sarcomas or carcinomas. Resistance commonly develops after prolonged use and can be drug-specific or across the alkylating class as a whole. Detoxification of these drugs by conjugation with nucleophilic glutathione prevents these drugs from interacting with DNA and increased levels of glutathione or elevated GST can be expected to result in resistance to all drugs within this class. Similarly, increased repair of DNA damage, as is seen with elevated levels of MGMT, can reduce sensitivity of cancer cells to all alkylating agents. Alternatively, resistance that is produced through reduced drug penetration into the cell can be drug specific; for example, melphalan is taken into the cell by the leucine transport system (Del Amo et al., 2008) whereas mechlorethamine is taken in by the choline transporter (Fry and Jackson, 1986) and altered transport affecting one of these drugs does not necessarily cause a reduced efficacy of the other. The most widely used nitrogen mustard in veterinary medicine is cyclophosphamide (Cytoxan, CTX) (Figure 44.2). Cyclophosphamide is a prodrug that must be converted by the hepatic mixed function oxidase system (cytochrome P450) to an intermediate compound, 4-hydroxycyclophosphamide, which then undergoes spontaneous degradation to the active compound phosphoramide mustard (Cohen and Jao, 1970). Because of the requirement of cytochrome P450 enzymes in the activation of cyclophosphamide, consideration must be given to potential drug–drug interactions in animals coadminstered either inhibitors or activators of these enzymes. The major dose-limiting toxicities in dogs and cats are myelosuppression and neutropenia with thrombocytopenia to a lesser degree. GI toxicity can also be of concern but is more commonly encountered in cats (Fetting et al., 1982). Cyclophosphamide, along with the other nitrogen mustards, is capable of producing alopecia in susceptible animals and causing loss of whiskers in cats. Sterile hemorrhagic cystitis is a potential toxicity that is encountered relatively infrequently and is more likely to develop in dogs than in cats. A metabolite of cyclophosphamide, acrolein, is thought to be responsible for irritation to the bladder following CTX administration. Intravenous administration of furosemide concurrently with cyclophosphamide has been shown to reduce the incidence of sterile hemorrhagic cystitis in dogs (Charney et al., 2003), and when given orally by owners it should be administered in the morning, water intake should be high, and the animal should be given ample opportunity to urinate throughout the day. Administration of MESNA (2-mercaptoethanesulfonate) has also been used prophylactically to bind and detoxify the urotoxic metabolites of cyclophosphamide and ifosfamide (West, 1997). If cystitis occurs with administration of cyclophosphamide the drug should be discontinued and another alkylating agent used in its place (such as chlorambucil). Although maximum plasma concentrations and overall exposure (AUC) to the parent molecule (CTX) are significantly lower when administered by the oral versus IV route, exposure to the active phosphoramide mustard is equivalent between the two routes in dogs (Warry et al., 2011). Thus, doses for oral and IV administration are the same. Figure 44.2 Chemical structure of cyclophosphamide, a nitrogen mustard alkylating agent. Cyclophosphamide is commonly used as part of multiagent protocols in the treatment of canine and feline lymphomas and as part of combination immunosuppressive therapy. Cyclophosphamide may also have utility in the treatment of canine malignant mammary gland tumors (Sorenmo, 2003). Dosing of cyclophosphamide in dogs ranges from 250 mg/m2 IV or oral bolus, to a more fractionated schedule of 50 mg/m2 daily for 3 days on a 3-week cycle (Lori et al., 2010). Low-dose cyclophosphamide (10–15 mg/m2/day) has also been investigated in solid tumors of dogs as part of a metronomic therapy protocol and has demonstrated some efficacy in that setting (Elmslie et al., 2008; Burton et al., 2011). Cyclophosphamide is available as 25- and 50-mg tablets and as a 25-mg/ml injection. Tablets should not be broken or split prior to administration. Ifosfamide (isophosphamide) (Figure 44.3) is a structural isomer of cyclophosphamide that, like cyclophosphamide, requires metabolic activation by hepatic P450 enzymes to form the bifunctional alkylating compound isofosforamide mustard (Creaven et al., 1974). The structural differences between cyclophosphamide and ifosfamide result in a reduced rate of the initial metabolism of ifosfamide to the active form. In addition, a secondary pathway of metabolism that generates chloroacetaldehyde results in the requirement of larger doses of ifosfamide when compared to cyclophosphamide (Wagner, 1994). Although ifosfamide is mostly indicated for treatment of sarcomas in animals, it has a relatively broad range of antitumor activity and has been evaluated in both canine and feline neoplasms. In dogs, a dose of 350 to 375 mg/m2 has been evaluated in a variety of tumor types with DLT of neutropenia occurring 7 days post treatment (Rassnick et al., 2000). In cats, the recommended starting dose of ifosfamide is 900 mg/m2 and has been evaluated in the treatment of vaccine-associated sarcoma with an overall response rate of 41% (Rassnick et al., 2006). The dose-limiting toxicity in cats is also neutropenia and the majority of cats tolerate ifosfamide treatment. Nephrotoxicosis is a potential adverse effect in cats treated with ifosfamide. Prophylactic therapy with saline diuresis and MESNA appear to be effective means to prevent sterile hemorrhagic cystitis following ifosfamide treatment and diuresis should be considered to reduce the risk of nephrotoxicosis in cats. The reason for the difference in the dose tolerated by dogs and cats has not been systematically evaluated but is likely to involve species differences in metabolism with regard to exposure to active and/or toxic metabolites. There is evidence of autoinduction of ifosfamide metabolism in humans with multiple fractionated doses resulting in increased levels of metabolites, which may result in increased toxicity (Kurowski et al., 1991; Kaijser et al., 1994), but this has not been evaluated in veterinary patients. As with most cytotoxic drugs, ifosfamide is most likely to provide the greatest benefit when used as part of a combination protocol. Ifosfamide is available in 1 or 3-gram vials for reconstitution and intravenous administration Figure 44.3 The nitrogen mustard alkylating agent ifosfamide, a structural isomer of cyclophosphamide. Mechlorethamine (mustargen), sometimes referred to as “nitrogen mustard,” was the first alkylating agent used clinically (Figure 44.4). Mechlorethamine is a bifunctional alkylating agent that is capable of forming interstrand DNA cross-links as a mechanism of cytotoxicity (Kohn et al., 1966). Unlike cyclophosphamide and ifosfamide, mechlorethamine undergoes spontaneous hydrolysis to form active nucleophilic compounds. Thus, interactions with drugs that inhibit or induce hepatic metabolism are not anticipated with mechlorethamine. Entry of mechlorethamine into cells appears to involve a carrier-mediated, active process and resistant cells demonstrate reduced entry of drug into the cell (Goldenberg et al., 1970). Structural analogs of mechlorethamine, such as cyclophosphamide, melphalan, and chlorambucil, do not appear to inhibit cellular uptake of mechlorethamine. In veterinary medicine, mechlorethamine is most commonly used in the treatment of relapsed or resistant multicentric canine lymphoma as part of the MOPP (mustargen, vincristine, procarbazine, prednisone) protocol (Rassnick et al., 2002; Brodsky et al., 2009; Northrup et al., 2009). Myelosuppression and gastrointestinal toxicity are the dose-limiting toxicities reported for mechlorethamine in combination protocols. The most commonly reported dose for mechlorethamine in dogs when used in combination protocols in 3 mg/m2 on days 0 and 7 of a 21-day cycle. Mechlorethamine is available as a lyophilized powder in 10-mg vials. Figure 44.4 Mechlorethamine, or mustargen, sometimes referred to as “nitrogen mustard” was the first alkylating agent to be used clinically. Chlorambucil (leukeran) (Figure 44.5) is an orally bioavailable alkylating agent that, along with the metabolite phenylacetic acid mustard, has direct bifunctional alkylating ability (Mitoma et al., 1977; Goodman et al., 1982). Chlorambucil is most often used in slower growing neoplasia such as chronic lymphocytic leukemia (CLL) but has been used in other types of cancers such as lymphoma and mast cell tumor (Taylor et al., 2009; Campbell et al., 2013). In cats, chlorambucil has been used in the treatment of GI lymphoma in combination with glucocorticoids, where it demonstrated favorable efficacy and was well tolerated (Stein et al., 2010). Metronomic therapy with chlorambucil has also been reported for a variety of neoplasms in dogs and may be a treatment option for dogs with transitional cell carcinoma of the urinary bladder (Leach et al., 2012; Schrempp et al., 2013). Chlorambucil has also been utilized as an alternative to cyclophosphamide when the latter has resulted in the development of sterile hemorrhagic cystitis. Chronic oral dosing in dogs with CLL generally begins with 3 to 6 mg/m2 daily for 1 to 2 weeks followed by a reduction to 3 to 6 mg/m2 every other day. Metronomic dosing studies have used a daily dose of 4 mg/m2 in dogs while a bolus dosing regimen of 20 mg/m2 every 2 weeks has been evaluated and found to be well tolerated in cats with GI lymphoma. The major dose-limiting toxicity for chlorambucil is myelosuppression with neutropenia and a lesser degree of thrombocytopenia. In humans, myoclonus has been reported as a rare side effect following both high- and low-dose treatment and myoclonus following chlorambucil treatment has also been reported in a cat (Benitah et al., 2003). Chlorambucil is available as 2-mg tablets for oral administration. Tablets should not be broken and animal doses should be adjusted to the nearest whole tablet. Figure 44.5 Chlorambucil, an orally bioavailable nitrogen mustard alkylating agent. Melphalan (alkeran) is a nitrogen mustard-containing alkylating agent (L-phenylalanine mustard) that has direct DNA alkylating properties (Figure 44.6). Structurally, melphalan is similar to chlorambucil yet it is charged and hydrophilic at neutral pH and thus requires active uptake by amino acid transporters, which can be blocked by the amino acid leucine (Del Amo et al., 2008). Melphalan is orally bioavailable with a relatively large percentage eliminated unchanged in the urine in humans (Tew et al., 2001). Melphalan is most commonly used for the treatment of multiple myeloma in dogs and cats where initial positive responses are frequent, although complete eradication of all neoplastic myeloma cells is unlikely and eventual relapse is anticipated (Matus et al., 1986). Melphalan has also been used as a rescue therapy for relapsed lymphoma in dogs (Alvarez et al., 2006; Back et al., 2013). Myelosuppression and, in particular, a delayed thrombocytopenia is the major dose-limiting toxicity for melphalan and monitoring of complete blood count (CBC), including platelet counts, should be performed every 2 weeks for the first 2 months of therapy, then monthly thereafter. Melphalan therapy appears to be more myelosuppressive in cats than in dogs (Hanna, 2005). Initial dosing of melphalan is 0.1–0.2 mg/kg by mouth daily for 7–10 days followed by a reduction to 0.05 mg/kg daily, continuously. Alternatively, a pulse-dosing regimen of 7 mg/m2 by mouth, daily for 5 days every 3 weeks has been utilized and is well-tolerated by dogs. In cats, long-term therapy consists of 0.1 mg/kg by mouth once every 7 days, continuously (Vail, 2013). Melphalan is available for oral use as 2-mg tablets and for intravenous injection as a 50-mg vial. Figure 44.6 Melphalan, structurally similar to chlorambucil, is charged and hydrophilic and requires active uptake into cells by amino acid transporters. Cyclohexylchloroethylnitrosurea (CCNU) is a monofunctional alkylating agent with a single chloroethyl group (Figure 44.7
Chemotherapy of Neoplastic Diseases
Terminology and Treatment Perspectives
Cancer Biology
Chemotherapy of the Cancer Cell
Tumor Cell Sensitivity
Tumor Cell Resistance
Mechanism
Drugs/classes involved
Reduced uptake
Methotrexate, gemcitabine, cisplatin, melphalan
Increased efflux
Doxorubicin, vinca alkaloids, taxanes
Decreased activation
Antimetabolites (nucleoside analogs)
Increased or decreased levels of drug target
Doxorubicin, methotrexate
Target mutations/reduced binding
Vinca alkaloids, methotrexate
Drug inactivation/detoxification
Alkylating agents, cisplatin
Increased damage repair
Alkylating agents, cisplatin, doxorubicin
Intracellular redistribution of drug
Doxorubicin
Transporter
Substrates
MDR1
Doxorubicin, vinca alkaloids (vincristine, vinblastine), taxanes, methotrexate, mitomycin C, epipodophyllotoxins
MRP1
Doxorubicin, vinca alkaloids, paclitaxel, mitoxantrone
MRP2
Cisplatin, vinca alkaloids, doxorubicin, mitoxantrone, methotrexate
BCRP
Mitoxantrone, camptothecin, methotrexate, etoposide, doxorubicina, methotrexatea
Combination Chemotherapy
Pharmacodynamic Response
Drug Toxicity
Specific Chemotherapeutic Drugs
Alkylating Agents
Nitrogen Mustards
Cyclophosphamide
Clinical use:
Ifosfamide
Mechlorethamine
Chlorambucil
Melphalan
Nitrosoureas
Cyclohexylchloroethylnitrosourea (lomustine)
Stay updated, free articles. Join our Telegram channel
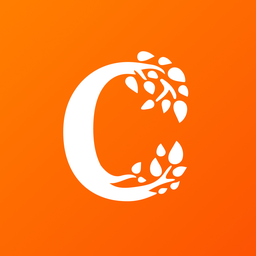
Full access? Get Clinical Tree
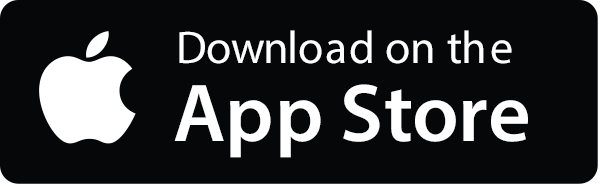
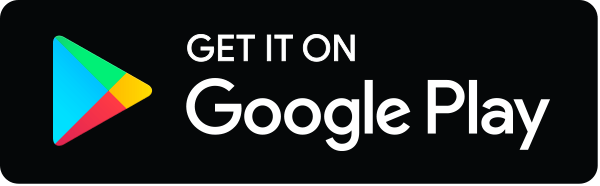