where M is the moment of the force, F is the force, and d is the moment arm. Moments of force are important in the effective function of muscle in sustaining weight bearing. For example, in the stifle the normal position of the patella creates an effective moment by the quadriceps about the center of rotation of the stifle such that extension of the stifle is maintained enough to bear weight. However, the function is altered when the patella is not in its normal position and is dislocated medially. In the dislocated position the moment arm of the tension force in the quadriceps is reduced or eliminated (Figure 24-1). The closer the tension force is to the center of rotation, the less extension is possible. Therefore a dog with luxating patellas is weak in the rear limbs and unable to bear weight normally or perform activities, such as jumping. A variety of levers are used by the animal to extend and flex the various joints of the limbs. For example, extension of the elbow is accomplished with a Class I lever in which the fulcrum is located at the center of rotation of the joint, the resistance or load force is located at the point where the foot contacts the ground, and the effort or action force, which is produced in the triceps muscle, is located at its insertion point on the olecranon (Figure 24-2). With flexion of the elbow, a Class III lever is in operation in which the effort is located at the insertion of the biceps brachii, which is between the fulcrum (elbow joint) and the resistance force, which is located at the end of the antebrachium (Figure 24-3). Class II levers are uncommon. An example is the rear foot, in which the weightbearing toes act as the fulcrum, the resistance or load force is the body weight just caudal to and at the foot, and the effort force is the gastrocnemius muscle (Figure 24-4). In the case in which rotation is inhibited by contracture or fibrosis leading to decreased ROM, therapists use “passive” ROM exercise by pushing or pulling on the lower part of the limb to induce flexion or extension in a target joint. Passive ROM is often used to reduce the possibility of stiffness or to improve the mobility of stiff joints. In some of these exercises the lever action is altered. For example, stretching in flexion of a dog with hyperextension stiffness of the stifle presents the conditions for a Class II lever (Figure 24-5). The point of resistance is the insertion of the quadriceps muscle on the tibial tuberosity, which is located between the fulcrum (stifle joint), and the effort, which is the location of the therapist’s hold on the distal crus of the leg. In this case, the effort is relatively far from the stifle when compared with the resistance that is close to the joint. When the quadriceps is adhered to the underlying bone and joint capsule and has lost its elasticity, it has also lost its capacity to absorb energy and load. As the therapist pushes on the tibia to produce flexion in the stifle, the resistance load in the stiff quadriceps quickly rises, creating a magnitude of force that has the ability to result in tearing of the quadriceps or even avulsion of the tibial tuberosity. Because the moment arm length of the effort force is great, a large moment can be generated, potentially causing damage. It is important for the therapist to understand the relationship between the effort or action load and the reactive or resistance load when employing certain therapeutic exercises. In the previous example, moving the hands closer to the stifle joint reduces the moment arm length, and therefore makes it more difficult to generate a large moment, reducing the possibility of tissue damage. Other exercises such as “wheel barrowing” on the front limbs or “dancing” on the rear limbs employ the use of lever systems. In the case of wheel barrowing or walking down an incline (to increase weight bearing on the front limbs), the spine becomes a Class II lever in which the resistance (center of gravity [COG] of the body) is located between the fulcrum at the shoulder joint and the effort in which the therapist is lifting the caudal end of the body. It is also important to note that as the spine is raised from the rear, the COG of the body moves to a location that is more dorsal to the shoulder, introducing a horizontal component of the weight, which acts as a shear force across the shoulder joint (Figure 24-6). As a result, increased stress in the joint capsule, collateral ligaments, and tendons will likely occur. This horizontal shear force may also cause an additional moment to occur at the level of the elbow joint, which is likely resisted by additional contraction of the triceps muscle. It has been observed that the angle of the shoulder joint becomes more extended with wheelbarrowing,1 which moves weight bearing from the center of the humeral head to a more cranial location where there is less articular cartilage and bone. Dancing is the reverse of this example; in dancing the spine is raised from the front and used as a Class II lever. As with the effects on the shoulders, the same likely occurs on the hips. For example, the addition of shear force across the hip joint has the potential of increasing mechanical stresses in the articular cartilage, which may be deleterious to a dysplastic joint. Increased moment across the stifle would likely be resisted by increased contraction of the quadriceps muscles, strengthening this muscle group. The effectiveness of physical rehabilitation exercises could be enhanced by detailed mechanical evaluation looking at not only the magnitudes of weight-bearing forces but also the altered direction of these forces. Stress is particularly important in the understanding of how articular cartilage responds to force applied across a joint surface. Under conditions in which the anatomy is normal and the joint is physiologically and mechanically normal, the surface of the joint spreads the applied forces over a wide area such that the stress at any specific point on the cartilage is low. However, if the joint is anatomically deranged, such as with a growth deformity, or is mechanically unstable, such as with hip dysplasia, joint forces are concentrated on smaller surface areas, causing high degrees of stress and subsequent breakdown of the articular cartilage (Figure 24-7). For any tissue under the influence of force, its response can be graphed as stress versus strain, which demonstrates additional properties of the tissue response to force (Figure 24-8). The initial response in the graph is termed the toe region of the graph. This phenomenon is more typical with soft tissue such as a tendon or ligament as opposed to bone. A tendon in its completely relaxed state has a crimped or wavy pattern of the collagen fibers. When the tendon is mounted in the testing machine and then pulled, the initial recording represents a straightening of the crimp and does not represent any part of the strength of the tendon in tension. It is also helpful to pass through this “toe” region by “prestressing” the tendon (i.e., stretching the tendon to eliminate the crimp). The slope of the graph gives a measure of stiffness of the tissue. Steep slopes occur when the tissue is inflexible and brittle like that of bone, whereas a shallow slope represents tissue with flexibility, like that of muscle or tendon. The linear portion of the graph describes the elasticity of the tissue. In this range the tissue is deforming or undergoing strain, but when the load is removed, the tissue returns to its original length. The upper curved portion of the graph describes the plasticity of the tissue. In this range the tissue is deforming, but when the load is removed the tissue does not return to its original shape, meaning that a permanent change has taken place; therefore the tissue has undergone a “plastic deformation.” The peak of the curve is the highest level of stress the tissue tested can withstand and is referred to as the ultimate strength of the tissue. Failure under stress is demonstrated as a decreasing capability to maintain the stress and the value begins to drop as the load increases, or complete failure as the stress drops to zero, indicating that the tissue can no longer hold up under any applied load. In addition to these features, the area under the stress versus strain curve represents the amount of energy that is absorbed or stored in the material as it is loaded. Once failure occurs, the stored energy is released in the form of a fracture or tearing event. where F is the resultant force, m is the mass, and a is the acceleration. Kinetic gait analysis can be used to evaluate normal weight bearing, identify alterations in weight bearing, aid in the diagnosis of disorders of locomotion, and evaluate treatment effects. The typical format for evaluating the forces involved in the motion of an animal is the use of the force platform. The force platform is a plate instrumented with a series of strain gauges or crystals and mounted into the floor. Because of their symmetry and convenient speed, the walk and trot are the conventional gaits that are used for evaluation of gait and assessment of lameness. The trot is usually the easier gait to obtain data. At a walk, dogs are more likely to be distracted and have a less consistent gait. When the animal steps on the plate, the sensitive strain gauges or crystals generate an electrical signal that is sent to a data processor and a computer. Multiple signals, collected over time, define the stance phase of the gait, from the time the foot strikes the plate until the instant the foot leaves the plate. The right side of the equation, the displacement side, is kept constant by setting the velocity and acceleration to a well-defined range, for example, trotting at a velocity of 1.7-2 m/s and acceleration of ± 0.4 m/s2. To generate a readable report, the animal is trotted across the plate such that the front and rear limb, on the same side, fully contact the plate once (Figure 24-9). Often an animal may not lead consistently or may be cautious of the plate by shifting weight, attempting to avoid contact. Therefore a single tracing is not considered reliable by itself. Several identical tracings (usually a minimum of three) are considered more reliable in presenting to the evaluator a more realistic representation of the actual gait routine for that animal. The force plate is capable of measuring reaction forces in all three dimensions: the vertical (z axis), the horizontal (y axis), and the transverse direction (x axis). Pressure pads or mats have also been developed and have the advantage of being able to evaluate patients too small to participate in force platform analysis and to collect data from multiple limb strikes. This may allow the objective evaluation of small dogs and cats with conditions such as avascular necrosis of the femoral head and medial patella luxation.2,3 However, they typically only measure relative weight bearing by a limb rather than actual force, and they do not allow the resolution to z, y, and x forces. Pressure exerted in the vertical direction on the force plate generates an equal but opposite reaction force in the force plate. This vertical GRF represents the animal’s weight-bearing performance and is often measured as a percent of body weight. The term peak force represents the maximum value of the GRF during the stance phase of gait, whereas impulse represents the generation or dissipation of reaction force occurring over time. Normal peak vertical GRF for the front limb at a trot is 110-125% of body weight, and for the rear limb is 65-75% of body weight,4 and are typically symmetric with a single peak (Figure 24-10). Walking generates a biphasic Z force curve similar to that seen in humans, resulting in a classic m-shaped graph in which the initial peak represents the vertical force component associated with the initial paw strike in the early stance phase and the second peak represents the increase in vertical force at the time of toe off or propulsion (Figure 24-11).5 Typical peak vertical force (Zpeak) measurements for the fore- and rear limbs while walking are lower than those measured while trotting and are typically 60% and 30% of body weight, respectively. Zpeak is affected by the gait, velocity, and acceleration of the dog, the dog’s body weight, conformation, and musculoskeletal structure.6–8 In both the walk and the trot, Zpeak increases as the forward velocity of the animal increases.6,9 Impulse, the area under the force-versus-time curve, is the change in momentum over time. As velocity increases at a trot, the vertical impulse (VI) increases as a result of the increased force.6,9,10 When comparing only peak vertical GRFs between individuals, how rapidly the limb is loaded and how long the limb bears the load are overlooked. These factors affect the impulse value and are not reflected in the peak force. If the limb bears load longer, for a similar peak load, the impulse will be significantly greater (Figure 24-12). ZPeak of a rear limb is greater while trotting than while walking, but because the stance time is longer at a walk, the impulse is greater while walking than while trotting. Therefore changes in both peak reaction force and impulse should be evaluated when rating gait performance, and repeated evaluations may only be compared if the dog is ambulating at the same gait, and velocity, and there is no acceleration or deceleration while measuring GRFs. The animal’s COG is particularly important in weight distribution at a stance or while in motion. Traveling uphill tends to shift the balance of forces toward the hindlimbs, and moving downhill shifts the forces to the forelimbs.8 These factors may affect the forces generated during muscle contraction and be used advantageously during strengthening programs. Further evaluation of kinetic information includes symmetry of the tracing, and comparisons of tracings to the contralateral limb and previous tracings. In cases of lameness, the peak vertical GRF and VI are significantly reduced. Coefficients of variation between trials range from 5% to 9% for Zpeak and VI, whereas variation is higher for braking and propulsive forces. Most of the variability is due to dog and trial variation, with little influence on variation by experienced handlers.11 The symmetry of the tracing can also be altered with lameness. If the shape of the tracing is shifted to the left or right, the animal is quickly loading the limb and peaking early in the stance phase and then slowly unloading the limb throughout the remainder of the stance phase, or slowly loading the limb and quickly unloading it. The clinical significance is not clearly understood regarding this shift, but one study has suggested that a combination of Zpeak and the falling slope (unloading the limb) is more valuable in assessing lameness caused by cruciate ligament disease than either measure by itself.12 Changes in the shape of the tracing could represent lameness because it is reasonable to surmise that a painful limb would not be allowed to increase its load all the way to midstance but would peak early, or the limb would be rapidly unloaded, when the limb pain reaches an intolerant level. However this shift has been seen in contralateral limbs that are compensating for lameness in other limbs. In the compensation mode, loading of the limb peaks early to reduce the load on the painful limb. The difference may be related to the Zpeak. In the case of the shift occurring with lameness, the Zpeak is less than normal, whereas in the case of compensation, the left shift in the compensating limb is normal or higher than normal. The stance time may also be assessed. The stance time of the gait cycle is the time when the limb is in contact with the ground and depends largely on the velocity of the subject. As the velocity increases, the stance time decreases.9,10 In addition, a lame limb typically has a shorter stance time compared with the unaffected limb.7,8,10,12 Pressure exerted in the direction of the animal’s motion occurs when the animal places the foot on the plate. This creates a GRF that is equal to the applied pressure but in the opposite direction. This GRF pushes back against the forward motion of the animal resulting in a braking action. As with the vertical force, the intensity of this braking force increases to a peak value then begins to decrease (Figure 24-13). However, there are important differences. Unlike the Zpeak, the braking force rises and falls in the first half of the stance phase, such that the horizontal GRF is zero at the midpoint of the stance phase. In addition, the braking force is opposite to the direction of animal motion and is therefore negative and appears below the horizontal axis. After the animal passes the midpoint of the stance phase, the pressure exerted by the foot on the plate causes a GRF that is in the same direction as the animal’s motion. This GRF pushes the animal along its motion and is therefore a propulsive force. Similar to braking, the propulsive force increases to a peak value and then decreases. The propulsive force cycles completely within the second half of the stance phase. These forces of braking and propulsion occur along the y axis. Normally, the forelimbs have greater braking forces than propulsive forces, and the hindlimbs have greater propulsion as compared with braking. Force plate and motion analysis systems are costly and require a relatively large dedicated workspace. Therefore their use in private practice is limited. Subjective lameness scales have traditionally been used, but have significant limitations. Weight bearing at a stance may be one method to obtain quantitative data regarding the relative amount of force placed on each limb while standing. A computerized system to objectively evaluate weight bearing at a stance in dogs may be useful for this purpose (Figure 24-14). Preliminary studies indicate that there is good correlation between Zpeak at a trot determined by force plate analysis and static weight-bearing pressures in dogs with rear-limb lameness.13 In fact, the difference between the lame and contralateral sound limb may be greater at a stance because there are three other limbs to shift the weight to, and the limbs are in contact for a longer time than at a trot, allowing additional time to shift the weight. Real-time photographic, optoelectric technology is effective in measuring angles during continuous motion. Optoelectric technology involves the use of infrared cameras positioned for views in three-dimensional space. The animal is prepared with reflective markers secured to the body at predetermined points (Figure 24-15). These points are anatomic features that represent joint anatomy. Infrared light emitted from individual cameras is reflected from these markers back to the camera (Figure 24-16). As the joints move, the cameras record the displacement of the markers. Data points are analyzed with respect to a three-dimensional grid set up and calibrated at the beginning of the test. Such a system can record joint angle excursion throughout a full stride. Typical data acquired include stride length, stance and swing times, joint angles in all planes of motion, and linear and angular joint velocity and acceleration. A stride includes a single swing and stance phase. The stance time of gait is the period when the foot is in contact with the ground, and the swing phase occurs when the foot is off the ground between stance phases. The swing phase has three distinct movements. The limb initially swings caudally following propulsion. The limb is then pulled cranially, and finally travels briefly caudally toward the ground in preparation for the next stance phase.14,15 The distance from initial contact of one limb to the point of second contact of the same limb is termed stride length, and a gait cycle is a series of events that includes one stride for each of the four limbs.5,14,15 The joint angle data can be plotted over the period of a single stride (Figure 24-17). These patterns of motion are specific for each joint and gait. Some results have been obtained both for normal gait and for lameness associated with cranial cruciate deficiency16 and hip dysplasia. where F is the force (kinetics: evaluation of force), m is mass, and a is acceleration (kinematics: evaluation of linear displacement). Specifically for angular motion (the study of the interior forces of the muscles and the angular movements of the bones): where Several studies have evaluated normal GRFs and kinematic motion of dogs at the walk and trot.4,6,7,9-12,14,17–27 Studies in normal dogs indicate that each joint has a characteristic and consistent pattern of flexion and extension during the walk, but complex joint movements may occur during the swing phase.14,19,27 Two additional studies described kinematic motion of the joints of normal dogs at a trot.17,18 The coxofemoral and carpal joints were characterized by a single peak of maximal extension and the femorotibial, tarsal, scapulohumeral, and cubital joints had two peaks of extension, with one peak occurring prior to the stance phase, and a second peak occurring during the stance phase.17,18 There were insignificant differences between trials and few differences among dogs of similar body type. It was concluded that kinematic gait analysis may provide a reliable description of joint motion in dogs of similar size and conformation. A recent study has evaluated not only the joint angle excursions at a walk, but also defined angular velocity and acceleration rates of those movements. This study indicated that these parameters are consistent and repeatable and helps to further characterize the normal walking gait of hound-type dogs.27 Additional studies are warranted to determine the sensitivity and specificity of alterations in these parameters as indicators for specific causes of lameness. Following cranial cruciate ligament rupture (CCLR), Zpeak may be only 50% of normal at a walk, and dogs may be non–weight bearing at a trot.28 By 7 months after extracapsular surgical repair, weight bearing is usually equal in both rear limbs at a walk. Experimentally, Zpeak at the trot with an extracapsular repair technique was normal by 20 weeks after surgery.29 Weight bearing in the contralateral rear limb initially increases, likely as a result of redistribution of weight from the affected limb to normal limbs, then returns to normal as weight bearing improves on the affected limb. Dogs with CCLR have variable degrees of lameness and demonstrate altered movement in the hip, stifle, and hock joints.16 The stifle joint angle in the cruciate-deficient state was more flexed throughout the stance and early swing phase of stride and failed to fully extend in late stance, when limb propulsion is typically developed. In addition, extension velocity was negligible. The hip and hock joint angles, in contrast, were extended more during the stance phase, perhaps as a result of compensatory changes.16 Paw velocity and stride length were also significantly reduced in dogs with CCLR walking on a treadmill as compared with the contralateral side, and to normal dogs.30 Changes in kinematics were evaluated over a 2-year period in dogs with CCL-deficient stifles.31 All changes occurred primarily between 6 and 12 months. Peak cranial tibial translation increased by 10 mm and the range of abduction and adduction motion nearly doubled (from 3.3 degrees to 6.1 degrees) after CCL transection and did not improve with time. These changes may overload secondary restraints such as the medial meniscus. This objective information further defines the pathologic gait of CCLR patients. More sophisticated techniques have been used to quantify net joint moments, joint powers, and joint reaction forces (JRFs) across the hock, stifle, and hip joints in Labrador retrievers with and without CCL disease using inverse dynamics calculations.32 Kinematic, GRF, and morphometric data were combined in an inverse dynamic approach to compute hock, stifle, and hip net moments, powers, and JRF while trotting. Vertical and braking GRF and JRF were decreased in CCL-deficient limbs. In fact, the braking/propulsion ratio shifted from 50% : 50% in normal limbs to 33% : 66% of the stance phase in CCL-deficient limbs. It is possible that reduced braking may result in decreased cranial tibial thrust. In affected limbs, extensor moments at the hock and hip, flexor moment at the stifle, and power in all three joints were less than normal. Greater joint moment and power of the contralateral limbs compared with normal were also identified. Other compensatory changes included increased time spent with the sound foot on the ground and an increased amount of force applied to all joints of the sound limb, especially during propulsion, compared with normal and CCL-deficient limbs. Propulsion generated around that joint at push-off was three times greater than normal, reflecting increased stifle extensor muscle contraction. Lameness predominantly affected forces during the braking phase and extension during push-off, and a greater contribution of the contralateral limbs to propel the dog forward was identified. These changes may be somewhat protective against damage to the articular cartilage and joint and may be mediated, in part, by the nervous system and a response to pain. In one study using a CCL transection and hindlimb deafferentation model, extension of the hip, knee, and ankle joints of the unstable limb was increased, and braking of the unstable knee was delayed or attenuated.33 These changes are different than models that have not used deafferentation. The model used in this study results in rapid onset and progression of osteoarthritis with cartilage erosions present by 13 weeks, perhaps because there is no pain sensation or joint proprioception is altered. Stifle hyperextension resulting from limb deafferentation, and knee instability resulting from CCL transection may work synergistically to create increased tibiofemoral motion and changes in the loading of articular surfaces that result in rapid joint breakdown.
Biomechanics of Physical Rehabilitation and Kinematics of Exercise
The Body in Equilibrium: Statics
Rotation (Moment of a Force or Torque)
Lever Systems in the Body (Application of Moments of Force)
Figure 24-2 Extension of the elbow is a Class I lever system. The fulcrum is located at the center of rotation of the joint, the resistance or load force is located at the point where the foot contacts the ground and the effort or action force produced in the triceps muscle is located at its insertion point on the olecranon.
Figure 24-3 A Class III lever occurs with flexion of the elbow where the effort is located at the insertion of the biceps brachii, which is between the fulcrum (elbow joint) and the resistance force, which is located at the end of the antebrachium.
Figure 24-4 An example of a Class II lever is the rear foot, where the weight-bearing toes act as the fulcrum, the resistance or load force is the body weight just caudal to and at the foot, and the effort force is the gastrocnemius muscle.
Figure 24-5 Stretching in flexion of a dog with hyperextension stiffness of the stifle presents the conditions for a Class II lever. The point of resistance is the insertion of the quadriceps muscle on the tibial tuberosity, which is located between the fulcrum (stifle joint), and the effort, which is the location of the therapist’s hold on the distal shin or crus of the leg. In this case the effort is relatively far from the stifle when compared with the resistance, which is close to the joint.
Figure 24-6 Wheelbarrowing to increase weight bearing on the front limbs results in the spine becoming a Class II lever in which the resistance (center of gravity of the body) is located between the fulcrum at the shoulder joint and the effort where the therapist is lifting the caudal end of the body. As the spine is raised from the rear, the center of gravity of the body moves to a location that is more dorsal to the shoulder.
Tissue Response to Force
Stress
Figure 24-7 In a mechanically unstable joint, such as in a dog with hip dysplasia, joint forces are concentrated on small surface areas, causing high degrees of stress and subsequent breakdown of the articular cartilage.
Stress versus Strain
Figure 24-8 A stress versus strain curve demonstrates additional properties of the tissue response to force. A, The initial response in the graph is termed the toe region of the graph. The collagen fibers are in a completely relaxed state with a crimped or wavy pattern. B, The linear portion of the graph describes the elasticity of the tissue. The slope of the graph gives a measure of stiffness of the tissue. C, The upper curved portion of the graph describes the plasticity of the tissue. In this range the tissue is deforming, but when the load is removed, the tissue does not return to its original shape, meaning that a permanent change has taken place. D, The peak of the curve is the highest level of stress the tissue tested can withstand and is referred to as the ultimate strength of the tissue.
The Body in Motion: Dynamics
Kinetics
Figure 24-9 The dog is trotting over the force platform, which is connected to a computer for the measurement of ground reaction forces.
Vertical Ground Reaction Forces
Figure 24-10 Vertical forces of the forelimbs and hindlimbs while trotting. Note that there is only a single smooth curve for the forelimbs and hindlimbs, with the greater forces placed on the forelimb.
Figure 24-11 Vertical forces of the forelimbs and hindlimbs while walking. Note the biphasic nature of the force curve.
Figure 24-12 The rate of limb loading and stance time affects the impulse value and is not reflected in the peak force. If the limb bears load longer, for a similar peak load, the impulse is greater.
Horizontal Ground Reaction Forces
Measurement of Static Weight Bearing
Kinematics
Figure 24-15 The animal is prepared with reflective markers secured to the body over anatomic features that represent joint anatomy.
Figure 24-16 Cameras that detect infrared light reflected back to the camera are used to capture the motion of reflective markers as the animal moves through the test area.
Combining Kinetics and Kinematics
is the moment (kinetics: evaluation of moment of force), I is the mass moment of inertia, and
is the angular acceleration (kinematics: evaluation of angular displacement).
Kinetic and Kinematic Gait Analysis Research
Normal Gait
Cranial Cruciate Ligament Disease
Stay updated, free articles. Join our Telegram channel
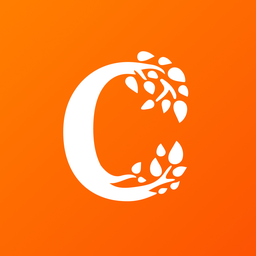
Full access? Get Clinical Tree
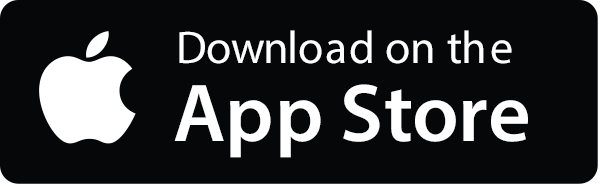
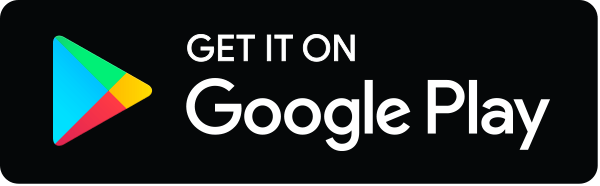