Extracorporeal shockwave therapy (ESWT) has been in use since the 1970s, when its original application was to provide a noninvasive means to fragment uroliths, allowing for subsequent passage of the small fragments through the urinary tract.1–3 Extracorporeal shockwaves have also been applied to the fragmentation of sialoliths, choledocholiths, and pancreatoliths.4 Applications to enhance bone healing began after observations of the effects of shockwaves on the pelvis during animal lithotripsy studies.5–7 These studies showed that a significant osteogenic response, specifically callous formation and bony remodeling, was elicited after exposure to extracorporeal shockwaves. The use of ESWT has also been investigated for the treatment of human musculoskeletal diseases such as pain associated with plantar fasciitis, lateral epicondylitis, calcifying tendinitis, and femoral head necrosis, as well as for the treatment of delayed union and nonunion fractures.1,8,9 Integration into treatment of soft tissue disorders, such as treatment of pain and erectile dysfunction associated with Peyronie’s disease or hypertonia secondary to stroke, has also gained favor.10,11 The use of ESWT in equine musculoskeletal disorders includes insertion desmopathies, bone spavin, tendon and ligamentous calcification, navicular disease, exostoses, fractures and microfractures, back pain, and osteoarthritis (OA) of tarsometatarsal and distal intertarsal joints. Extracorporeal shockwaves have been used in dogs as a part of the treatment regimen for hypertrophic nonunions, tendonitis, spondylosis, and OA4–6,12 (Figure 22-1). Extracorporeal shockwaves are acoustic waves of high pressure and velocity produced outside of the body. These waves are characterized by high-amplitude acoustic pressures (20-100 megapascals [MPa]) with a short build-up time of approximately 5-10 nanoseconds, an exponential decay to baseline to a negative deflection of approximately 10 MPa, and a wave cycle time of 300 nanoseconds (Figure 22-2).2,6,13,14 These pressure waves differ from ultrasound waves because of their lower frequency, minimal tissue absorption, and lack of a thermal effect.2 Focused generators produce pressure waves in a fluid medium when electrical energy is converted to mechanical energy. These waves may be focused by reflection of the pressure waves within the generator to the focal point, which is at the treatment site (Figure 22-3).6,13 The focused waves behave as sound waves in the tissues, releasing energy when tissue density changes are encountered (i.e., ligament to bone interface).3,6 At the interface of density change, part of the wave is transmitted while the other part is reflected.7 The greater the change in tissue density, the greater the energy released. Three types of focused shockwave generators exist: electrohydraulic, electromagnetic, and piezoelectric. Piezoelectric generators use crystals that expand and deform when stimulated by high-voltage electricity, initiating a pressure wave.2,7,13 The electromagnetic system consists of coils arranged in such a way as to generate opposite magnetic fields when high voltage electricity is applied.2,7 When a high current pulse is released through the coil, a strong magnetic field is generated, creating a high current in the opposing metal membrane. The membranes are forced away from each other as a result of the opposing currents, causing compression of the surrounding fluid medium, resulting in a shockwave.2,7,13 Electrohydraulic generators use a high-voltage spark gap, causing plasma bubble generation. Expansion of the bubble results in sonic pulse propagation and subsequent collapse results in a reverse pulse, manifesting a shockwave (Figure 22-4).2,7,13 Delivery of energy to tissue requires the use of coupling gel in conjunction with a percussive handheld applicator. Energy can be focused via spherical arrangement of crystals, spherical generator, or acoustic lenses. Focusing energy results in greater tissue depth penetration, up to 110 cm, with a rapid decline of energy at the periphery, allowing an intense amount of energy delivery to a relatively small area.6,13 Eighty percent of the maximum shockwave pressure resides in the focal area. Hence, its maximum value is in the focus with a decrease in pressure at the periphery of the focal area.4 Piezoelectric generators have a small focal volume with high-energy flux values and low overall energy transfer. Electromagnetic generators also have a small focal volume and high-energy flux, but tend to be less concentrated than the piezoelectric system. Electrohydraulic generators produce the largest focal volumes with lower energy flux density and overall high energy transfer.13 An alternative to focused extracorporeal shockwaves exists as a radial extracorporeal shockwave device, or a ballistic generator. Shockwaves from this device are generated pneumatically (mechanical concussion) and are propagated through tissues in a radial pattern. Radial pressure waves have lower energies, characterized by a slower rise time, and a negative component that is the same magnitude as the positive component.15 The energy applied by this device is greatest at the applicator-skin interface and decreases proportionally to the square of the distance from the applicator head, applying energy to a wider circumference with decreased tissue penetration.12,13,15–17 The rapid release of energy occurs over a relatively wide area with a rapid decrease in energy delivered to deeper tissues.5,6,12 The primary effects of shockwaves are produced by the generation of mechanical forces. Secondary effects occur by indirect generation of mechanical forces through cavitation. Compression and tension generation occurs as the shockwave travels through the tissue. Varying amounts of reflection and transmission of energy occur at varying interfaces. In bone, for example, the amount of energy attenuation depends on the amount of cancellous bone, because attenuation of the shockwave increases exponentially with increased bone thickness.9 This is largely due to the various tissue interfaces; hence most of the energy from the shockwave is deposited in cancellous bone.2,6 Cavitation bubbles are made during the tensile phase of the shockwave, and as size increases, a large amount of energy is delivered to the bubble. Cavitation bubbles formed secondary to shockwave-generated compression and tension forces have the potential for deposition of large amounts of energy after subsequent collapse, releasing high-energy water jets and high-temperature production.3,7 Cavitation at the surface interface is the mechanism by which fragmentation of uroliths occurs.3 Cavitation also leads to the production and release of free radicals, ultimately creating chemical reactions within the tissue. ESWT may also increase cell membrane permeability. The growth and collapse of cavitation bubbles, which may lead to surface deformation and damage is one potential mechanism of action for increasing membrane permeability.18 A dose-dependent cytotoxicity or decrease in cell viability is present with increased numbers of waves and energy density. Different cell populations differ in their sensitivity to shockwave energy. Bone marrow, for example, has a decreased sensitivity compared with malignant cell lines.19 Human fibroblasts have an increased sensitivity to higher numbers of shockwaves rather than the higher energy of shockwaves, decreasing the growth potential of fibroblasts while in suspension.20 The reepithelialization rate of skin wounds in pigs is also affected by ESWT, and the effects depend on the energy level used.4,21 The mechanism of action for induction of analgesia and tissue healing is poorly understood. Human patients tend to exhibit a bimodal analgesic effect, in which an initial decrease in pain 3-4 days after initial treatment has been attributed to the direct effects of shockwaves on nociceptors and impaired synthesis of substance P.22 After the initial analgesic effects, a gradual recurrence of pain is noted with a subsequent gradual resolution of pain noted over 3-4 weeks that is attributed to angiogenesis and tissue matrix remodeling.22 Analgesic effects in humans appear to be more consistent in chronically affected patients rather than acutely affected patients.23 Horses have also exhibited bimodal analgesia; however, the mechanism remains unknown.13 It has been hypothesized that analgesia is induced by hyperstimulation of nociceptors resulting in an intense sensory input to the periaqueductal gray area of the midbrain. This sensory input promotes descending inhibition and modulation of sensory stimulus transmission through the dorsal horns of the spinal cord, known as the “Gate Control Theory.”15,24,25 A second theory is that induction of cell damage from shockwave application prevents appropriate membrane potentials required for transmission of signals.15 It has been shown that shockwaves induce production of cytokines and growth factors, endothelial nitric oxide synthase (eNOS) and up-regulation of bone morphogenetic protein (BMP) expression.26–28 As a result of cytokine and growth factor release, a decline in inflammation and swelling, with short-term analgesia, is noted. Decreases in calcitonin gene–related peptide (CGRP) in free nerve endings and dorsal root ganglia (DRG) of rats after application of ESWT to the plantar skin of the hindlimb support the theory that altered nerve function of the locally treated area may be the source of analgesic effects.29,30 Substance P gained interest as a mode of analgesia, as an increase in basal concentration was noted 24 hours after ESWT application to the femur of a rabbit, followed by a gradual decrease in basal secretion over 6 weeks. This decrease was attributed to a potential degeneration of nerve endings in the treated area.31 Later studies by Haake and colleagues,32–34 did not find evidence of ESWT influence on spinal antinociceptive opioid mechanisms or on nonopioid mechanisms (substance P or CGRP). ESWT did not appear to activate peripheral nerves to induce analgesia, as evidenced by a lack of increase in c-Fos protein or its gene expression.32 Local desensitization and subsequent reinnervation has been theorized by Murata and colleagues,35 in which an increase in activating transcription factor 3 and growth-associated phosphoprotein 43 was measured in rat DRG neurons after treatment with ESWT. Although a definitive mechanism of action and therefore treatment protocol for analgesia still remains to be elucidated, Takahashi and colleagues36 described a cumulative effect of shockwaves on nerve fibers on the skin of rats, suggesting that multiple applications of low-energy shockwaves may provide longer-lasting antinociceptive effects. McClure and colleagues25 and Abed and colleagues37 investigated analgesia induced by focused or radial shockwaves. Twenty-four horse limbs were assigned to receive control (sham treatment), ESWT, or radial pressure wave treatment (RPWT). Analysis of cutaneous analgesia revealed a substantial increase in cutaneous threshold to electrical stimuli for ESWT and RPWT at day 3. Analysis of neuropeptide concentrations, substance P, and CGRP did not reveal a significant difference between the groups.25,37 Sensory nerve conduction velocity was found to be statistically lower in nonfocused radial pressure waves when compared with control sensory nerve conduction. Disruption of the myelin sheath in medium- to large-diameter axons was noted under transmission electron microscopy, potentially creating a prolonged conduction velocity in radial pressure wave–treated nerves.15 There is tremendous interest in evaluating ESWT as a treatment for joint conditions, such as OA. Mueller and colleagues8 reported on the analgesic benefit of radial ESWT for hip OA in dogs, characterized by improved ground reaction force difference between limbs 4 weeks following shockwave therapy and a potential long-term benefit of at least 3 months following treatment. Results of analgesia were similar to a study of canine stifle OA, in which three shockwave treatments (200 shockwaves were applied to each of four sites using a 20-mm focused depth applicator, followed by 175 shocks to each of the same four sites using a 5-mm focused depth applicator, at an energy flux density of 0.14 mJ/mm2, for a total of 1500 shocks) were administered 3 weeks apart. An improvement in peak vertical force and vertical impulse was evident at 21 days and continued to improve to the end of the study, at 98 days. Despite these improvements, the changes were not statistically significant from control groups perhaps because of small numbers of animals in each group.1 In contrast, Francis and colleagues38 reported significant improvement in ground reaction forces of dogs with elbow or hip OA when compared with control groups. A follow-up study of another group of dogs with elbow OA confirmed these findings and suggested that the amount of improvement with ESWT is similar to what would be expected when treating with an average nonsteroidal antiinflammatory drug (NSAID), despite the fact that the dogs were already receiving treatment for their arthritis, including NSAIDs.39 Several studies have investigated how ESWT may affect the processes involved in OA. One study evaluated the effects of ESWT on the metabolism of human OA chondrocytes in vitro, especially the expression of β1 integrin, interleukin (IL)-10, and tumor necrosis factor alpha (TNF-α).40 Human articular cartilage was obtained from patients with knee OA undergoing total joint replacement. After isolation, chondrocytes underwent ESWT with different parameters of impulses, energy levels, and energy flux density. Chondrocyte intracellular IL-10 and TNF-α levels were evaluated. At baseline, osteoarthritic chondrocytes expressed significantly higher levels and IL-10 and TNF-α levels. Following ESWT, there was a significant decrease of IL-10 and TNF-α intracellular levels. IL-10 levels decreased with any number of impulses and energy levels, whereas TNF-α decreased at middle energies. ESWT significantly reduced the nitric oxide level in the synovial cavity of knee joints in a rabbit anterior cruciate ligament (ACL) transection model as compared with untreated rabbits with cruciate ligament transection.41 Chondrocyte apoptosis and the severity of cartilage lesions were also less in rabbits with OA treated with ESWT. The authors concluded that ESWT may be a useful treatment for knee OA because of the reduced progression of OA in rabbits. Although promising, further studies are necessary to determine if ESWT may modulate the changes seen with OA. Another study evaluated the effects of ESWT on the development of OA in a rat ACL transection OA model.42 OA of the knee was detected radiographically 12 weeks after study initiation in the ACL-transected group, but very subtle changes were noticed in the control nontransected knees and the ACL-transected plus ESWT groups (single treatment with 800 shocks, 0.18 mJ/mm2). The articular cartilage of ACL-transected rats showed significant increases in cartilage degradation and chondrocyte apoptosis and significant decreases in subchondral bone remodeling compared with the control and ACL-transected plus ESWT rats. In addition, the ACL-transected plus ESWT rats had significantly decreased cartilage degradation and increased chondrocyte activity comparable to nontransected controls. The authors concluded that ESWT may have chondroprotective effects and improved subchondral bone remodeling in this rat OA knee model. In fact, ESWT of the subchondral bone may result in greatly reduced progression of OA.43 Another study by the same group also showed that ESWT reduced cartilage changes in a rat OA model, with the most beneficial effects seen 4 weeks after ESWT. The group receiving ESWT had significantly lower histologic changes, increased collagen type II in the articular cartilage, and increased vascular endothelial growth factor (VEGF), BMP-2, and osteocalcin in the subchondral bone compared with the untreated rats.44 Studies in a horse OA model, however, indicated no effect on subchondral bone, although increases in the serum biochemical markers osteocalcin and C-terminal telopeptide indicated bone remodeling.45 Clinical function and the pain of OA may also be modulated by ESWT. In a rat OA model, walking duration on a treadmill was significantly extended 4, 7, and 14 days after ESWT in rats with knee OA, but began decreasing by day 21.46 Although OA resulted in increased expression of CGRP in DRG neurons innervating the knee as compared with normal rats, ESWT reduced CGRP-positive DRG neurons, suggesting that ESWT is a useful treatment for knee OA. Effects of ESWT on articular cartilage are of some concern, however, because if disruption of the structure of cartilage occurs secondary to ESWT application, joint health may be compromised. Gross disruption of cartilage integrity was absent after radial shockwave application to equine third metacarpal or metatarsal bone.16 A dose-dependent increase in lactate dehydrogenase after radial shockwave application supported the thought of increased chondrocyte membrane permeability.16 Focused shockwave effects on articular cartilage have also been investigated and these studies indicated a lack of radiographic, histologic, and macroscopic changes within joint cartilage of growing rabbits.47 In vitro investigation of effects of focused shockwaves on articular chondrocytes revealed a dose-dependent increase in cytotoxicity, as well as decreased proliferative potential at higher energy doses.48 Another study evaluated a single bout of ESWT (1500 shockwaves of 0.5 mJ/mm2) on the structural integrity of articular cartilage of rat femoral heads in vivo.49 Although there were no changes in histologic scores after ESWT, positive immunostaining for tenascin-C and chitinase 3–like protein 1 was found up to 10 weeks after ESWT in treated cartilage and collagen type II mRNA was increased 1 and 4 weeks after ESWT. Other changes included expansion of the rough-surfaced endoplasmic reticulum, detachment of the cell membrane, and necrotic chondrocytes. Because all of these changes have been associated with early OA, there is some concern that high-energy ESWT directly applied to cartilage might cause degenerative changes in hyaline cartilage. In collagenase-induced models of suspensory ligament desmitis, destruction of abnormal metaplastic tissue and increased numbers of collagen fibrils was noted on histologic examination, suggesting possible promotion of healing of desmitis with ESWT.2,50 Evaluation of the bone-tendon interface in dogs and rabbits has revealed increased neovascularization after treatment, as indicated by an increase in neovessels and angiogenic markers, such as vascular endothelial growth factor, eNOS, and proliferating cell nuclear antigen expression.51,52 In a collagenase-induced patellar tendinopathy model in rabbits, increased tensile strength, hydroxyproline concentration, and tenocyte numbers, in addition to increased neovascularization, were reported.53 In a rat model of collagenase-induced Achilles tendonitis, low-energy ESWT induced repair coinciding with an increase in transforming growth factor beta1 (TGF-β1) and insulin-like growth factor I (IGF-I) concentrations with tenocyte proliferation, neovascularization and progressive tissue regeneration, whereas higher energies impaired DNA, extracellular matrix synthesis, and biomechanical characteristics of tendons.26 Increases in TGF-β1 expression were noted in early tendon repair stages and IGF-I expression was increased throughout the healing process, indicating that TGF-β1 and IGF-I are important mediators in ESWT-stimulated cell proliferation and tissue regeneration within a healing tendon.26,50 An increase in metachromasia was also noted and may be an indication of increased extracellular matrix production by cells.50,54 Increases in glycosaminoglycan and protein synthesis have been seen as early as 3 hours after ESWT application, with an overall tendency toward anabolic metabolism. Acceleration of healing may therefore be attributed to an initiation of ESWT-induced stimulation to the tissue with a subsequent decrease in cellular metabolism over 6 weeks.55 Increased edema and inflammatory cells in the paratenon of rabbits has been described and could indicate that although ESWT has the potential to treat various tendinopathies via induction of tissue regeneration, there is also the potential for harm of tendinous tissue with application of ESWT.55–57 Documented changes appear to be dose dependent, either by the number of impulses or higher energy flux densities, and may decrease the mechanical strength, making these structures susceptible to tearing.58 The amount of energy and number of pulses delivered should be taken into consideration, because tendons and ligaments appear to be more susceptible to damage from excess energy.54–57 Radial pressure wave application has been investigated for the treatment of various tendinopathies, including insertional tendinopathies, with treatment successes reported in up to 80% of treated patients.59,60 In a study evaluating shockwave therapy for the treatment of supraspinatus calcifying tendinopathy, one dog exhibited subjective and objective improvement in lameness 21 days after shockwave therapy.61 Another dog in the same study was bilaterally affected and improvement was noted at 28 and 49 days after treatment in the right limb but not in the left limb. In both cases, disruption of the calcified material was not apparent after treatment. ESWT has also been evaluated for the treatment of patellar ligament desmitis in dogs after undergoing tibial plateau leveling osteotomy (TPLO).62 Dogs that had TPLO surgery were evaluated by radiographs and ultrasonography preoperatively and 4, 6, and 8 weeks after TPLO. At 4 and 6 weeks, treated dogs received 600 shocks with an energy level of 0.15 mJ/mm2, with a focused depth of application of 5 mm on the patellar ligament. There was a significant difference in distal patellar ligament thickness between groups at 6 and 8 weeks postoperatively. No significant ultrasonographic differences were found.
Applications of Extracorporeal Shockwave in Small Animal Rehabilitation
How Shockwaves Are Generated
Figure 22-2 Pressure profile of an extracorporeal shock wave. Note the rapid increase to a relatively high pressure, followed by a rapid decline. (Courtesy of Pulsevet, Inc.)
Figure 22-3 A trode used to apply extracorporeal shockwaves to a focused tissue depth. (Courtesy of Pulsevet, Inc.)
Delivery of Energy
Research Regarding Extracorporeal Shockwave Therapy
General Biologic Effects
Analgesia
Cartilage and Chondrocytes
Tendon and Ligaments
Stay updated, free articles. Join our Telegram channel
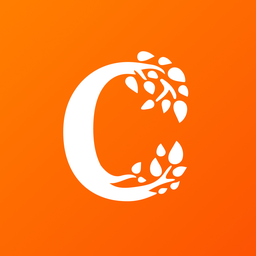
Full access? Get Clinical Tree
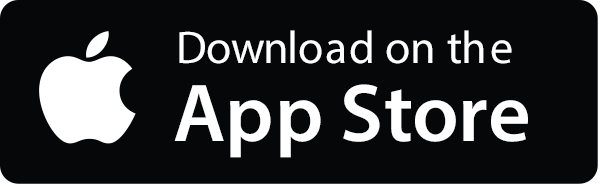
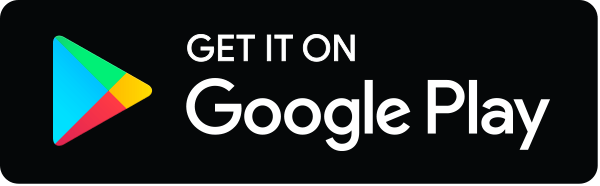