39 Carlos E. Lanusse, Juan M. Sallovitz, Sergio F. Sanchez Bruni, and Luis I. Alvarez The economic importance of helminth infections in livestock has long been recognized and it is probably for this reason that the most important advances in the chemotherapy of helminthiasis have come from the animal health area (Horton, 1990). Anthelmintics are used in all animal species and man. A significant part of the economic impact of parasitism in animal production is represented by the investment in control measures. Although alternative methods have been developed, chemically based treatments are the most important tool to control parasitism. A more complete understanding of the pharmacological properties of existing antiparasitic drugs should assist with more efficient parasite control both in livestock and companion animals. However, the investment in control measures does not always result in the expected therapeutic success. Among factors responsible for that therapeutic failure are: (i) inadequate integration between management strategies and chemotherapy; (ii) incorrect use of anthelmintic drugs due to insufficient knowledge of their pharmacological features; and (iii) insufficient understanding of the relationship between pharmacological properties and several host-related factors that could lead to modifications of the pharmacokinetic behavior and to a decreased antiparasite efficacy of the chosen drug. In addition, the availability of many compounds with a common mode of action and the indiscriminate use of these drugs have accounted for the widespread development of drug resistance, mainly in parasites of sheep and goats, but also in parasites of pigs, horses, and cattle. This chapter covers the information available for the different specific antinematodal drug families used in veterinary therapeutics. However, special consideration is given to the description of the large body of pharmacological knowledge generated for the benzimidazole compounds, the most intensively studied anthelmintic chemical group together with the endectocide macrocyclic lactones (see Chapter 41). Benzimidazoles (BZD) and pro-benzimidazoles (pro-BZD) anthelmintics are widely used in veterinary and human medicine. The pro-BZD are inactive prodrugs metabolically converted into anthelmintically active BZD molecules in the host. The remarkable overall safety of BZD compounds has been a major factor in their successful worldwide use over four decades. BZD were introduced into the animal health market primarily for the control of gastrointestinal (GI) nematodes, not only for use in livestock animals (cattle, sheep, goats, swine, and poultry), but also for horses, dogs, and cats. The use of BZD compounds quickly became widespread because they offered major advantages over previously available drugs in terms of spectrum, efficacy against immature stages, and safety for the host animal. Since the discovery of thiabendazole in 1961 (Brown et al., 1964), several thousand BZD molecules have been synthesized and screened for anthelmintic activity. However, no more than 20 of them have been commercially developed for use in domestic animals and man, either as BZDs or pro-BZDs. The BZD structure is a bicyclic ring system in which benzene has been fused to the 4- and 5- positions of the heterocycle (imidazole) (Figure 39.1). Most of the BZD compounds are white crystalline powders, with fairly high melting point and insoluble or slightly soluble in water. Their aqueous solubility is markedly higher at low acidic pH values, with the stomach/abomasum being the appropriate site for the dissolution of BZD drug particles after oral treatment. The BZD compounds can be grouped as follows: Figure 39.1 Chemical structures of the main benzimidazole (BZD) anthelmintics used in veterinary medicine. The positions 2- and 5- (main substitution sites) are shown in the thiabendazole structure. (#) ABZSO is also known as ricobendazole (RBZ). Triclabendazole (a halogenated flukicidal BZD) is not included here (see Chapter 40). Different modifications at positions 2- and 5- of the BZD ring system (see Figure 39.1) have provided the most anthelmintically active drugs, especially, with the discovery of sulfur-containing methylcarbamate derivatives such as albendazole and fenbendazole, which exhibit high efficacy against lungworms and inhibit larval stages of most GI nematodes. The BZD methylcarbamates are the most used BZD compounds. Albendazole, fenbendazole and their sulfoxide derivatives (albendazole sulfoxide and oxfendazole, respectively) (ruminants), fenbendazole and oxfendazole (horses), febantel, fenbendazole, and mebendazole (companion animals), fenbendazole and flubendazole (poultry and pigs) are currently among the most extensively used BZD methylcarbamate anthelmintics in veterinary medicine. Microtubules are hollow tubular organelles that exist in a dynamic equilibrium with tubulin, the microtubule subunit. Tubulin is a dimeric protein comprised of α- and β-subunits. The BZD and pro-BZD pharmacological activity is based on the binding to parasite β-tubulin, which produces subsequent disruption of the tubulin–microtubule dynamic equilibrium (see Lacey, 1990 for detailed information). Competitive binding experiments using tubulin from mammalian, invertebrate, or fungal cells indicate that BZD compounds bind within the colchicine (a well recognized microtubule inhibitor) binding domain on tubulin. Thus, all the functions ascribed to microtubules at the cellular level are altered (cell division, maintenance of cell shape, cell motility, cell secretion, nutrient absorption, and intracellular transport) (Lacey, 1988). Microtubules are found in animals, plants, and fungi cells. However, the rate constant for the dissociation of BZD from parasite tubulin is much lower than the rate constant for the dissociation from mammalian tubulin. These differences in dissociation rates between BZD and tubulin in host and parasites may explain the selective toxicity of BZD compounds to parasites and its wide safety margin in the mammalian host. The microtubule loss observed at tegumental and intestinal level in cestodes and nematodes after BZD treatment is followed by loss of transport of secretory vesicles and decreased glucose uptake. A prolonged storage of secretory material within the cells is followed by cell disintegration. Cell autolysis requires a period of 15–24 hours posttreatment. Additionally, the inhibition of secretion of nematode acetylcholinesterase and inhibition of some enzymatic activities (such as fumarate reductase, malate dehydrogenase, phosphoenol pyruvate reductase, and succinate dehydrogenase) have been associated with the BZD anthelmintic action. However, all these effects may be related to the primary underlying BZD mechanism: the disruption of the tubulin–microtubules dynamic equilibrium. The effects of structural modifications to BZD-like molecules on microtubule inhibitory activity have been intensively investigated (reviewed by Lacey, 1988). It has been postulated that the presence of a carbamate group in the 2- position is essential for potent microtubule inhibitory activity. Additionally, regardless of the size of the substituent in the 5- position, the pharmacological effect heavvily depends on the nature of the molecule adjacent to the BZD ring system. Different studies suggest that not only the chemical substitution in the position 5- of the BZD ring, but also its conformational arrangement, are relevant in the access of the drug to the active site and in the resultant anthelmintic activity. The anthelmintic activity of BZD compounds not only depend on its binding to β-tubulin, but also on their ability to reach high and sustained concentrations at the site of parasite location, which allow the delivery of effective concentrations of the compound at the receptor within the parasite cells, in sufficient time, to cause the therapeutic effect (Thompson et al., 1993). As a chemical class, the BZD methylcarbamates have only limited water solubility and small differences in drug solubility may have a major influence on their absorption and resultant pharmacokinetic behavior. The lack of water solubility is an important limitation for the formulation of BZD compounds, which mainly allows their preparation as suspensions, pastes, or granules for oral or intraruminal administration. Poor/erratic GI absorption is a common inconvenience for the systemic availability of enterally administered BZD suspensions in most species. The mucous surface in the GI tract behaves as a lipid barrier for the absorption of active substances, so that absorption depends on lipid solubility and degree of ionization at GI pH levels. However, drug particles must dissolve in the enteric fluids to facilitate absorption of the BZD molecule through the GI mucosa. A drug that does not dissolve in the GI contents, passes down and is excreted in the feces without exerting its action. The BZD methylcarbamates show only limited GI absorption due to their poor solubility in water. Their dissolution rate, passage along the GI tract, and absorption into the systemic circulation, are markedly slower than those observed for the more hydrosoluble BZD thiazolyls (TBZ, thiabendazole). Such a phenomenon also results in extended residence times for the active metabolites of the BZD methylcarbamates compared to those of TBZ. This differential pharmacokinetic behavior accounts, in part, for the greater anthelmintic potency of fenbendazole and albendazole compared with thiabendazole. The complexity of the ruminant digestive tract in comparison to that of the monogastric animal creates unique problems and opportunities related to the absorption of drugs administered orally. The rumen may substantially influence the absorption pattern and the resultant pharmacokinetics and antiparasite activity of enterally delivered BZD anthelmintics. The rumen accounts for about 20% of the animal’s volume and never empties. When a BZD suspension is deposited in the rumen, solid particles mix and distribute through the digesta volume. Shortly after administration, BZD compounds are almost completely associated (adsorbed) to the digesta particulate material. The drug reaches an equilibrium between the particulate and fluid portions of the digesta. The drug dissolved in the GI fluid is available for absorption and/or for diffusion through the external surface of the helminth parasite located at the GI lumen. The adsorption of BZD particles to digesta solid content, the slow mixing and long digesta residence time, and the large rumen volume assists absorption by delaying the rate of passage of drug down the GI tract (Hennessy, 1993). The rumen acts as a drug reservoir by slowing the digesta transit time throughout the abomasum, which results in improved systemic availability of BZD compounds as a consequence of a greater dissolution of drug particles in the acid pH of the abomasum (Lanusse and Prichard, 1993a). The plasma levels of the parent sulfides (i.e., albendazole, fenbendazole) and/or its active sulfoxide metabolites (albendazole sulfoxide, oxfendazole) reflect the amount of dissolved drug at the GI level (Figure 39.2). Since the main mechanism of drug entry to nematode parasites located in the GI lumen is drug diffusion through its external surface, the higher the concentration of solubilized drug in the GI fluid, the greater the anthelmintic activity. The influence of the rumen on BZD absorption is evidenced by the higher and more sustained concentration of fenbendazole, oxfendazole, albendazole, and their metabolites recovered in the bloodstream after oral/intraruminal treatments compared to intraabomasal administration of the same compounds in sheep (Prichard et al., 1978; Marriner and Bogan, 1981). Figure 39.2 Schematic representation of the main pharmacokinetic features taking place after oral/intraruminal administration of benzimidazole anthelmintics in ruminants. See the text for further explanation. Albendazole (ABZ) is used as a model drug in the scheme. A lack of proportionality in the relationship between albendazole dose rates and drug systemic exposure has been described in sheep, where area-under-the-curve (AUC) and maximum peak concentration (Cmax) values for albendazole sulfoxide increased more than expected from the increase in the dose. The enhanced systemic exposure achieved after albendazole treatments at the highest dose rates correlated with significant increment in drug efficacy against a resistant Haemonchus contortus strain (Alvarez et al., 2012). BZD and pro-BZD anthelmintics are extensively metabolized in all mammalian species studied. As a common pattern among different BZD compounds, the parent drug is short lived and metabolic products predominate in plasma and all tissues and excreta of the host, as well as in parasites recovered from BZD-treated animals (Fetterer and Rew, 1984; Alvarez et al., 1999, 2000). The primary metabolites usually are products of oxidative and hydrolytic processes and are all more polar and water soluble than the parent drug. In addition, phase II conjugative reactions are highly important in the detoxification of BZD-derived products. The oxidized and hydrolyzed metabolites are conjugated with glucuronide and/or sulfate to increase their polarities, which facilitates urinary or biliary excretion. Since BZD anthelmintics are mainly administered by the oral route, “first-pass” metabolism is relevant in the kinetic behavior of these compounds. Intestinal, liver, and lung metabolism have been implicated in this phenomenon in most animal species. Additionally, GI metabolism is an important concern in ruminant species. Netobimin (pro-BZD) is an anthelmintically inactive nitrophenylguanidine prodrug. Netobimin is nitroreduced and cyclized into albendazole in a microflora-mediated reductive reaction in the GI tract (Lanusse et al., 1993a). The nitro-reduction and cyclization of netobimin into albendazole in the host are crucial for the pharmacokinetic profile of its active metabolites and resultant anthelmintic activity. Both formulation and route of administration may dramatically affect the rate of netobimin conversion and the bioavailability and disposition of its main plasma metabolites, albendazole sulfoxide and albendazole sulfone. Sheep and cattle ruminal and ileal fluids can convert netobimin into albendazole under in vitro conditions (Lanusse et al., 1992b). However, the subcutaneous administration of netobimin resulted in lower plasma concentrations of active metabolites compared to those measured after oral administration of the prodrug in sheep and cattle (Lanusse et al., 1990). In conclusion, oral administration results in an improved pharmacokinetic profile of netobimin metabolites, which accounts for some advantageous anthelmintic efficacy after the oral/intraruminal compared to the parenteral treatment. Febantel is a phenylguanidine prodrug, which is hydrolyzed by removal of a methoxyacetyl group and then cyclized to fenbendazole. Fenbendazole is then converted to oxfendazole and fenbendazole sulfone in subsequent oxidative metabolic steps. Following febantel administration, in both sheep and cattle, the parent drug is not found in plasma, or is detected in low concentrations for only a short period. In contrast to the extensive GI metabolism described for netobimin, only a low proportion of febantel is bioconverted by sheep and cattle ruminal fluids (Beretta et al., 1987). Hepatic metabolism appears to be the main site of febantel conversion into fenbendazole, after its oral administration to different animal species. The active molecule of the pro-BZD thiophanate is an ethyl BZD carbamate metabolite known as lobendazole (Delatour et al., 1988), formed in the liver with conversion rates of 34% (sheep), 52% (goats), and 57% (cattle). Thiophanate is currently used as an antifungal agent (thiophanate-methyl) for plants. The metabolism of BZD heavily depends on the substituent present at position 5- of the BZD ring system and involves a variety of reactions. Phase I reactions have been observed at position 5-. Hydroxylations of thiabendazole, albendazole, and fenbendazole have been demonstrated in different animal species. The sulfoxidation of albendazole and fenbendazole at the sulfur atom of the substituent group at the carbon 5- of the BZD nucleus have been widely investigated. The oxidations of albendazole to albendazole sulfoxide and fenbendazole to oxfendazole have been shown to be catalyzed by the liver microsomal mixed function oxidases in different species (Galtier et al., 1986a; Souhaili El Amri et al., 1987; Montesissa et al., 1989; Lanusse et al., 1993b; Virkel et al., 2004). Additionally, the anthelmintically active sulfoxide derivatives undergo a second, slower and irreversible oxidative step, forming the inactive sulfone (albendazole sulfone and fenbendazole sulfone) metabolites, which are also found in the bloodstream and tissues after administration of their respective parent sulfides. The substitution of the BZD ring in position 5- has been particularly important in determining the metabolic fate of BZD methylcarbamates. This position is metabolically labile and has permitted retardation of the biotransformation of 5-substituted BZD anthelmintics as well as improvement of their efficacy (Hennessy et al., 1989). The nature of this substitution at position 5- markedly influences the sequence of BZD liver metabolism. Aromatic BZD derivatives, such as fenbendazole and oxfendazole, require more extensive hepatic oxidative metabolism than aliphatic derivatives (albendazole and albendazole sulfoxide) to achieve sufficient polarity for excretion (Hennessy, 1993). Consequently, the rates of albendazole liver microsomal sulfoxidation in sheep and cattle were higher than those observed for fenbendazole (Virkel et al., 2004). For this reason, low fenbendazole concentrations are recovered in plasma following its oral/intraruminal administration to sheep and cattle, whilst albendazole is not detected in the bloodstream after its administration as the parent drug in both species. Moreover, longer plasma mean residence times and elimination half-lives for fenbendazole and its metabolites, compared to those of albendazole metabolites, were observed in sheep and cattle (Lanusse et al., 1995) (Figure 39.3). Figure 39.3 Comparative mean (n = 5) plasma concentrations of the benzimidazole sulfoxide metabolites, albendazole sulfoxide (ABZSO) and oxfendazole (OFZ), obtained after oral administration of albendazole (ABZ), fenbendazole (FBZ), and OFZ (5 mg/kg) to adult sheep (see the text for further explanation). MRT, mean plasma residence time (h) for each molecule. Source: Adapted from Lanusse et al., 1995. Reproduced with permission of John Wiley & Sons. The involvement of the cytochrome P450 (CYP) and flavin monooxygenase (FMO) systems on the liver sulfoxidation of albendazole has been demonstrated in different animal species, including sheep (Galtier et al., 1986a) and cattle (Lanusse et al., 1993b). It has been demonstrated that FMO-mediated sulfoxidation accounted for up to 60% of albendazole sulfoxide production from albendazole, whilst CYP contributed 40% in both sheep and cattle liver microsomes (Virkel et al., 2004) (Figure 39.4). Similarly, FMO was estimated to be the main enzymatic system involved in the liver sulfoxidation of fenbendazole (∼80%) in both species (Virkel et al., 2004). Inhibition of CYP-mediated sulfoxidation by piperonyl butoxide demonstrated the participation of this enzymatic system in the hepatic metabolism of fenbendazole in horses (McKellar et al., 2002). Figure 39.4 Proposed metabolic pathways for the enantioselective sulfoxidation and sulfonation of (a) albendazole (ABZ) and (b) fenbendazole (FBZ). The gastrointestinal (GI) sulforeduction of both sulfoxide enantiomers (ruminants) to form the parent thioethers (ABZ, FBZ) is also shown. The width of the arrows represents the magnitude of each metabolic pathway (see the text). FMO, flavin monooxygenase; CYP, cytochrome P-450; ABZSO, ABZ sulfoxide; OFZ, oxfendazole, FBZ sulfoxide; ABZSO2, ABZ sulfone FBZSO2, FBZ sulfone. Source: Data from Delatour et al., 1991; Benoit et al., 1992; Redondo et al., 1999; Virkel et al., 2002, 2004. Both sulfoxide metabolites (albendazole sulfoxide and oxfendazole) have an asymmetric center in the sulfur atom of their side chains. This nucleophilic sulfur atom is attached to four different functional groups, which results in an asymmetric molecule nonsuperimposable with its mirror image. Thus, two different enantiomers of albendazole sulfoxide and oxfendazole have been identified (by chiral separation) in the plasma of sheep and cattle treated with albendazole and fenbendazole (prochiral molecules), respectively (Delatour et al., 1990a; Sánchez et al., 2002). It has been shown that (+)albendazole sulfoxide is the main enantiomeric form recovered in plasma and tissues at parasites locations (Alvarez et al., 2000) following albendazole treatment of sheep (Figure 39.5) and cattle. The same pattern was observed after albendazole sulfoxide administration to cattle (Cristofol et al., 2001). Most importantly, the (+)albendazole sulfoxide enantiomer seems to have a higher anthelmintic activity than that observed for the (−) enantiomer (Paredes et al., 2013). Similarly, (+)oxfendazole prevails in the systemic circulation after both fenbendazole and oxfendazole administrations to sheep (Delatour et al., 1990a; Sánchez et al., 2002) and cattle. The observed differences in the plasma/tissues availabilities of the (+) and (−) enantiomeric forms were attributed to the relative contribution of the FMO and CYP-dependent oxygenases to albendazole and fenbendazole hepatic sulfoxidation. FMO and CYP are known to be oppositely enantioselective (Cashman, 1998). FMO activity accounted for 94% (cattle) and 81% (sheep) of (+)albendazole sulfoxide production in liver microsomes (Virkel et al., 2004). In both species, the enantioselectivity of the hepatic FMO system towards (+)albendazole sulfoxide production was close to 100%. Moreover, it has been shown that (−)albendazole sulfoxide, rather than its (+) antipode, is the main substrate for the CYP-mediated formation of the inactive sulfone metabolite (Delatour et al., 1991; Benoit et al., 1992). On the other hand, FMO-mediated sulfoxidation of fenbendazole generated both (+) and (−)oxfendazole enantiomers. Fenbendazole oxidation was enantioselective towards the production of (+)oxfendazole in both sheep (65%) and cattle (79%). In conclusion, the sulfoxide metabolite recovered in plasma and target tissues after either albendazole or fenbendazole administration exists as two different chemical entities: the (+) and (−) enantiomeric forms (Figure 39.4). Figure 39.5 Schematic representation of the ruminal biotransformation of albendazole sulfoxide (ABZSO) enantiomers. The width of the arrows indicates the magnitude of the metabolic reactions. The main pharmacological features of the (+) sulfoxide enantiomer (compared to the (−) isoform) are listed in the bottom box. The enantioselective ruminal sulforeduction of the (+) isoform is the most relevant metabolic reaction for the formation of the parent thioether (ABZ). The same pattern is applicable to the sulforeduction of oxfendazole (OFZ) enantiomers into fenbendazole (FBZ) (see the text for detailed explanation). Source: Adapted from Virkel et al., 2004. Reproduced with permission of the American Society for Pharmacology and Experimental Therapeutics. Biotransformation takes place predominantly in the liver, although metabolic activity is apparent in extrahepatic tissues such as lung parenchyma and small intestine mucosa. Large quantities of albendazole parent drug were recovered from tissues at parasite locations in both sheep and cattle. Similarly, fenbendazole has been recovered from tissues at parasite locations following oral administration of oxfendazole to cattle. Altogether, these findings support the need to study the biotransformation of BZD anthelmintics in extrahepatic tissues such as lung parenchyma and small intestinal mucosa. The sulfoxidation of albendazole and fenbendazole by small intestine (cattle) and lung (sheep and cattle) microsomes was shown to be enantioselective (Virkel et al., 2004). Although the liver is the main site of albendazole and fenbendazole biotransformation, sulfoxidation in the intestinal mucosa and lung tissue may contribute to the presystemic metabolism of both anthelmintic drugs and should not be underestimated. The administration of thiabendazole results in a rapid conversion of a parent compound into a 5-hidroxy thiabendazole metabolite, formed by aromatic ring hydroxylation. Although this metabolite is sufficiently polar for a rapid urinary excretion, both its unconjugated and sulfate or glucuronide conjugated forms have been found in urine of TBZ treated animals (Tocco et al., 1965; Gottschall et al., 1990). After the administration of mebendazole to sheep, the parent drug is rapidly absorbed and metabolized into two main metabolites, which are identified in sheep plasma after mebendazole treatment (Behm et al., 1983). The main mebendazole metabolite results from carbonyl reduction to the secondary alcohol, which was also identified as its glucuronide or sulfate conjugates. Although, the specific enzymatic system responsible for mebendazole reduction is unknown, several cytosolic ketone reductases are involved in the formation of hydrosoluble metabolites from carbonyl-containing molecules. On the other hand, the combination of carbonyl reduction and carbamate hydrolysis produces the hydrolyzed metabolite of mebendazole. Hydrolysis of the carbamate group eliminates both the anthelmintic activity of the compound and its toxicity. Mebendazole rather than its metabolites appears to be the active anthelmintic molecule. Mebendazole metabolites are mainly excreted in bile. Unlike aromatic substituted methylcarbamates, 5-aliphatic substituted such as albendazole and parbendazole, when oxidized, are sufficiently polar to be largely excreted in urine rather than undergo further conjugation and secretion in bile (Hennessy et al., 1989). For instance, following albendazole treatment to sheep only 8% of the total dose was recovered in bile as unconjugated albendazole sulfoxide and hydroxyalbendazole sulfoxide metabolites, and 6.3% as conjugated glucuronide and sulfate esters, mainly of 2hydroxy-albendazole sulfoxide and 2hydroxy-albendazole sulfone (Hennessy et al., 1989); 59% of the dose was recovered in urine of albendazole-treated cattle (Gyurik et al., 1981). On the other hand, the percentage of a fenbendazole dose in cattle recovered in urine (2.5%) was markedly lower than that recovered from feces (42%), the latter being mainly the unchanged fenbendazole parent compound (Short et al., 1987). The parent flubendazole compound and its reduced and hydrolyzed metabolites have been identified in tissues of treated pigs, poultry, and sheep. After flubendazole intravenous administration to sheep, the parent drug was rapidly depleted from the bloodstream (Moreno et al., 2004), being detectable up to 36 hours posttreatment. The reduced metabolites (main metabolite) and hydrolyzed metabolites were rapidly detected in the bloodstream, which indicated a fast biotransformation of flubendazole to form both metabolic products. In vitro incubations with sheep liver microsomes indicated that flubendazole is converted to reduced flubendazole by the mixed-function oxidase system (Moreno et al., 2004). In comparison to the liver, where oxidative metabolism predominates, the GI microflora is very active in reductive reactions of foreign compounds, particularly those containing -nitro and -sulfoxide groups (Renwick et al., 1986; Rowland, 1986). Drug GI metabolism may be modified by the type of diet and many other factors affecting the GI bacterial reductive capacity. Orally administered compounds that are poorly absorbed from the GI tract will stand the greatest chance of undergoing metabolism by the microflora, although a large number of drug/metabolites gain entry to the gut via biliary secretion or plasma–GI distribution exchange (Figure 39.2) and will also be exposed to microbial activity. Drug metabolic processes taking place in the rumen are particularly important in ruminant therapeutics. The metabolic sulforeduction of the BZD sulfoxide metabolites (albendazole sulfoxide and oxfendazole) to form the parent thioethers (albendazole and fenbendazole, respectively) has been shown to occur in ruminal and intestinal fluid contents from sheep and cattle (Lanusse et al., 1992b). A plasma–GI pH gradient (Lanusse et al., 1993a) and/or an active gastric secretion process (Alí and Hennessy, 1995) account for the distribution of BZD metabolites from plasma to the digestive tract lumen. Therefore, albendazole sulfoxide and oxfendazole can be reduced back to their respective thioethers by ruminal and intestinal microflora and may act as a source of albendazole and fenbendazole, respectively, in the GI tract. This GI metabolic reduction may be of primary importance for the antiparasitic efficacy of BZD thioethers. Since the thioethers have a greater affinity for parasite tubulin than the sulfoxides (Lacey, 1990; Lubega and Prichard, 1991), this bacteria-mediated reduction may have significant importance for the efficacy against GI parasites. Thus, the high efficacy of albendazole sulfoxide and oxfendazole against GI parasites may depend on this bacterial reduction of the sulfoxide to the more pharmacologically active thioethers. The ruminal sulforeduction of both albendazole sulfoxide and oxfendazole is enantioselective, the (+) antipode being the main substrate to form the more active parent sulfide in the rumen (Virkel et al., 2002) (Figure 39.5). Chiral inversion is the metabolic process by which one enantiomer is transformed into its antipode. The chiral inversions of (+) into (−), but also from (−) into (+) enantiomeric forms, were observed when both enantiomers were incubated separately (as pure substrates) with ruminal fluid obtained from both sheep and cattle (Virkel et al., 2002) (Figure 39.5). Thus, the reported data suggest that chiral inversion of albendazole sulfoxide and oxfendazole enantiomers is likely to be bidirectional and may depend on either the metabolic transformation of one enantiomer into its antipode or through the formation of parent thioether (albendazole or fenbendazole) as an intermediate metabolic product. Overall, the work published by Virkel et al. (2002) demonstrates that the ruminal sulforeduction of both albendazole sulfoxide and oxfendazole is enantioselective, the (+) antipode being the main substrate to form the more active parent sulfide in the rumen. Release from the dosage form and absorption precede entry of any anthelmintic molecule into the bloodstream, which serves as the tissue in which drug and metabolite molecules are conducted to various parts of the body. Within the bloodstream, a fraction of most drugs binds reversibly to plasma proteins, and the remainder undergoes simultaneous distribution, metabolism, and excretion. The access of BZD molecules to intracellular sites depends upon their ability to penetrate the capillary endothelium and to cross the cell membrane. Most anthelmintics are weak organic acids or bases and exist in solution, at physiological pH, as both nonionized and ionized forms; while the poor lipophilicity of the ionized molecules excludes them from passive diffusion, the lipid-soluble nonionized moieties passively diffuse across biological membranes until equilibrium is established. Once the BZD molecule has been absorbed from the GI tract, it is rapidly distributed by the circulatory system throughout the entire body. During this time, the metabolic processes necessary to facilitate its elimination commence. Extensive tissue distribution of albendazole and its metabolites in sheep (Alvarez et al., 1999) and cattle (Sánchez et al., 1997) have been reported. However, differential distribution patterns among BZD sulfides (albendazole, fenbendazole), sulfoxide (albendazole sulfoxide, oxfendazole), and sulfone (albendazole sulfone, fenbendazole sulfone) metabolites, based on their differential lipophilicities, may be expected. The distribution rate, which is indicated by the apparent volume of distribution, depends on molecular weight, lipid solubility, and plasma protein binding of each drug/metabolite. The majority of BZD compounds show a binding of less than 50% to plasma protein, relatively high volume of distribution, and a relatively fast elimination rate in ruminant species. The rate of absorption, metabolism, and excretion of BZD anthelmintics varies from drug to drug, with slower absorption and prolonged recycling between enteral and parenteral tissues being relevant to enhance efficacy. Worms attached to the lining of the gut may be more exposed to this recycling drug than to that actually passing down the GI tract in food that is being digested. Fenbendazole, oxfendazole, and albendazole are less water soluble than earlier members of the group and, therefore, their dissolution rate, passage along the GI tract, and absorption into the systemic circulation are markedly slower compared to thiabendazole. These more lipophilic substituted BZD methylcarbamate compounds remain in the bloodstream for a longer time and, since it is assumed that an equilibrium exists between plasma and GI tract, the period of exposure of GI nematodes to effective drug/metabolites concentrations is extended. In NTB-treated cattle, peak concentrations of albendazole sulfoxide and albendazole sulfone are reached at 7–10 hours (albendazole sulfoxide) and at 15–22 hours (albendazole sulfone) posttreatment, followed by a well-defined decline in concentration in both plasma and GI compartments. However, whereas plasma concentration fell to undetectable levels (30–36 hours posttreatment), the profile of these metabolites in the rumen, abomasum, and ileum showed an “extra” slow elimination phase that extended to 72 hours posttreatment (Figure 39.6). This pharmacokinetic behavior is particularly clear for the albendazole sulfoxide and sulfone metabolites, whose elimination half-lives in both the abomasum and ileum were significantly longer compared to plasma. This metabolite-distribution process, also described for fenbendazole metabolites in cattle, may be driven by a plasma/GI tract pH gradient. The ratio of nonionized to ionized forms depends upon the pKa of the drug and the pH of the fluid in which the drug is dissolved, and the pH gradient between plasma and different tissues dictates the concentrations of drug/metabolite at either side of the separating cell membranes; at equilibrium, there will be a higher total concentration of the drug on the side of the membrane where the degree of ionization is greater. For instance, albendazole sulfoxide is an amphoteric compound which can act as either acid or base. Its pKa values are 3.45 and 9.8 for the acidic and basic groups, respectively. At plasma pH, there will be an important proportion of this molecule under the nonionic form, which will facilitate its passive diffusion from plasma to parasite location tissues. A greater plasma/abomasum pH gradient compared with that of the rumen and ileum would produce a strong ionic trapping effect (Figure 39.6), which would account for the significantly higher concentrations of albendazole metabolites found in the abomasum in comparison with plasma and other GI compartments. In addition, although albendazole (the most potent anthelmintic molecule) is not detected in the bloodstream, it has been recovered in different GI compartments, with particularly high concentration profiles recovered in the abomasal mucosa as well as from Haemonchus contortus recovered from treated sheep (Figure 39.7). The extensive distribution of BZD methylcarbamates from the bloodstream to the GI tract and other tissues may contribute to anthelmintic efficacy against parasites localized in body tissues including GI mucosa, GI lumen, and lungs (Alvarez et al., 1999, 2000). The recovery of both albendazole and fenbendazole parent compounds from GI mucosa and lung tissue after intravenous administration of their sulfoxide derivatives to cattle (as described in Section Reductive Metabolism in the Gastrointestinal Tract), is further evidence of the complex relationship among drug distribution, metabolism, and efficacy of BZD anthelmintics in ruminants. Figure 39.6 Comparative albendazole sulfoxide (ABZSO) concentration profiles measured in the bloodstream and in different gastrointestinal compartments after the oral administration of netobimin prodrug (20 mg/kg) to cattle. The results shown the slow elimination phase observed in digestive compartments (compared to plasma), which is critical for efficacy against gastrointestinal nematodes (see the text for further explanation). ABZSO elimination half-life values were: 2.29 hours (plasma), 16 hours (rumen), 34 hours (abomasum), and 67 hours (ileum). Source: Adapted from Lanusse et al., 1993a. Reproduced with permission of John Wiley & Sons. Figure 39.7 Comparative albendazole (ABZ) and albendazole sulfoxide (ABZSO) availabilities (expressed as area under the concentration vs. time curve, AUC), obtained in plasma, abomasal fluid, abomasal mucosa, and Hemonchus contortus recovered from infected sheep treated with ABZ (intraruminal at 7.5 mg/kg) (see the text for further explanation). ND, not detected. AUC values are expressed in µg.h/g. Source: Data from Alvarez et al., 2000. Comparative studies have revealed considerable differences between ruminant species regarding the pharmacokinetics of anthelmintic drugs. Longer residence times for albendazole sulfoxide in sheep than in cattle, and higher plasma AUC ratios for albendazole sulfone/albendazole sulfoxide in cattle, have been consistently reported. Since sheep liver microsomes has a higher albendazole sulfoxidase activity compared with that of cattle, the greater capacity of sheep GI fluids to reduce albendazole sulfoxide back to albendazole may explain the observed species-related pharmacokinetic differences. On the other hand, the oxfendazole AUC after oxfendazole administration to goats was 39% smaller compared to that observed in sheep (Hennessy et al., 1993a). It is suggested that goats possess a faster hepatic metabolism than sheep, resulting in more rapid elimination of oxfendazole. This lower availability may account for the poor efficacy reported for oxfendazole given to goats at the dose recommended for sheep. These observations suggest that extrapolated data can be misleading in predicting adequate dosages and withdrawal times. The oral administration of BZD (fenbendazole, oxfendazole) compounds in horses demonstrated that reduced bioavailability and shorter residence times are obtained in comparison to those observed in ruminants. Following oral administration of either fenbendazole or oxfendazole (10 mg/kg) to horses, the inactive fenbendazole sulfone metabolite is the predominant analyte measured in plasma, with AUC ratios oxfendazole/fenbendazole/fenbendazole sulfone of approximately 3/1/9 (oxfendazole treatment) and 1/4/7 (fenbendazole treatment) (McKellar et al., 2002). In both cases, the time to reach the peak plasma concentration of the active oxfendazole metabolite was approximately 9 hours, which is earlier compared to that observed in ruminants (21–29 hours) and agrees with the reduced systemic availability observed in horses. Following oxibendazole oral administration to horses (10 mg/kg), parent oxibendazole could only be detected in plasma up to 1 hour posttreatment; an unidentified metabolite was recovered in the bloodstream between 0.5 and 72 hours (Gokbulut et al., 2002). Oxibendazole in vitro conversion into its metabolite was significantly inhibited by coincubation with the cytochrome P450 inhibitor piperonyl butoxide. These findings indicate that a first-pass metabolism may reduce oxibendazole systemic availability in horses. As described for ruminant species, albendazole is not detected in plasma after its oral administration to pigs (Alvarez et al., 1995). Albendazole sulfoxide and albendazole sulfone are the main metabolites recovered from plasma up to 48 hours posttreatment. The pattern of plasma metabolites is similar to that reported after the administration to ruminant species and there appear to be no major differences between the metabolic patterns of albendazole observed in pigs and sheep. However, marked differences in the metabolism of albendazole between cattle and pigs have been described, with predominance of the sulfoxide metabolite over albendazole sulfone in pig plasma, while the inverse is observed in cattle after albendazole treatment. Similar to the observations in ruminants, the time of residence of the albendazole suspension at the acidic pH of the stomach in the pig affects its dissolution rate and the subsequent absorption of the drug from the gut. Any factor that influences gastric emptying rate may have a profound effect on the rate and extent of BZD absorption in monogastric animals. After oral fenbendazole administration to pigs, oxfendazole (the main metabolite recovered) and fenbendazole sulfone are rapidly detected in plasma up to 48 hours posttreatment. Oxfendazole reaches a Cmax value (0.7 µg/ml) at 12.5 hours posttreatment (Petersen and Friis, 2000). The bioavailability of fenbendazole in pigs after its oral administration was estimated to be about 30%. This low value is a consequence of the low water solubility of fenbendazole and its poor GI absorption, plus the significant liver first-pass effect on the drug. Interestingly, fenbendazole was measured in the bloodstream of oxfendazole-treated pigs. Its presence could be explained by oxfendazole reduction, which probably takes place in the pig large intestine, where an important microbial activity is present, as it is the main site of cellulose degradation in this species. The gut transit time influences the dissolution and absorption of poorly water-soluble drugs such as the BZD anthelmintics. The drugs that do not dissolve in GI contents pass down and are excreted in the feces without exerting their action. The GI physiology and the digesta transit time are markedly different between monogastric species and ruminants. This is relevant for dogs and cats, whose GI transit times are significantly shorter compared to ruminant species; this has a marked effect on the absorption, kinetic behavior, and efficacy of BZD anthelmintics in companion animals. Albendazole, albendazole sulfoxide, fenbendazole, febantel, or mebendazole, formulated as tablets or suspensions for oral administration in dogs and cats, must dissolve at low pH (stomach), and it has been demonstrated that the dissolution rate may be altered according the size of the formulation particle (Hennessy, 1993). Several studies suggest that only limited rates of dissolution and absorption of BZD anthelmintics are achieved in the cat, dog, and man. Consequently, these compounds may need to be given at a higher dose or as multiple administrations in order to maintain therapeutic concentrations and, therefore, to achieve acceptable anthelmintic efficacy (Roberson and Burke, 1982; Edwards and Breckenridge, 1988). Mebendazole is poorly absorbed after oral administration and only low concentrations (representing 10% or less of the total administered dose) of mebendazole parent drug and its inactive metabolite, hydroxy-mebendazole, were recovered in plasma of humans and dogs after oral treatment (Witassek et al., 1981; Edwards and Breckenridge, 1988). This observation could explain the lack of efficacy of mebendazole against lung parasites in humans and dogs and the need for multiple dose treatments. The pharmacokinetics of fenbendazole parent drug and its metabolites in dogs has been characterized (McKellar et al., 1990). Fenbendazole parent drug, its active sulfoxide (oxfendazole), and inactive sulfone (fenbendazole sulfone) metabolites were recovered in plasma for 48 hours after administration of a single oral dose of 20 mg/kg. Interestingly, plasma concentrations for both active molecules (fenbendazole and oxfendazole) are depleted in parallel from the body, giving an AUC ratio (fenbendazole : oxfendazole) of approximately 0.82. This may result in exposure of target parasites to the fenbendazole moiety (which has greater potency than oxfendazole), accounting for some advantageous activity against the tissue-arrested larvae and other immature parasite stages (McKellar et al., 1990). When albendazole was given as a single dose in tablet form, albendazole sulfoxide and albendazole sulfone were the main metabolites detected in plasma for up to 16 hours posttreatment (Sánchez et al., 2000a). Compared to ruminants, where the rumen acts as drug reservoir, effective treatment in monogastrics requires more prolonged, multidose regimes of at least 3 to 5 days, depending on the dose used. There are conclusive pharmacokinetic results to support the concept that higher efficacy could be expected in dogs and cats by increasing the number of treatments rather than increasing the dose. In fact, the AUC of fenbendazole in dogs was similar after a single administration of fenbendazole at different dose levels over a range between 2.5 and 100 mg/kg (McKellar et al., 1993). Lower fenbendazole plasma concentrations were achieved in dogs when fenbendazole was given on an empty stomach, compared with its administration given in food. However, fat content in the diet does not affect the absorption of fenbendazole after its oral administration to dogs (McKellar et al., 1993). After the oral administration of fenbendazole to chickens, the parent drug, oxfendazole and fenbendazole sulfone, were detected in plasma, fenbendazole sulfone being the main metabolite recovered up to 96 hours posttreatment (Taylor et al., 1993). A new formulation albendazole sulfoxide–base (ricobendazole) has been marketed for use in dogs in Latin America. Interestingly, this formulation showed around 500% increase in AUC and Cmax values when compared to the conventional albendazole tablet formulation (Dib et al., 2011). This increased systemic exposure correlated with enhanced efficacy against most GI helminth parasites, from which this formulation is recommend as a single dose treatments. The improved efficacy obtained at a single (20 mg/kg) dose may be based on the high solubility of albendazole sulfoxide at the different digestive tract pHs compared to albendazole parent drug. Albendazole parent drug is absorbed slowly and detected between 1 and 6 hours posttreatment in chickens, albendazole sulfoxide being the main metabolite detected, with a peak concentration of 0.83 µg/ml obtained at 1 hour posttreatment (Csiko et al., 1996) and low albendazole sulfone concentrations measured between 2.5 and 30 hours after albendazole administration. Interestingly, while in other animal species, such as pigs, dogs, and ruminants, albendazole is not detected in plasma after its oral administration, the parent drug was measured in hens. This finding has been associated with a fast absorption process and/or a slow metabolic rate in hens compared with other species (Bistoletti et al., 2013). Additionally, while drug absorbed at either proventriculus, gizzard, or intestines reaches the liver drained by the venous portal system, albendazole absorbed at the crop reaches the systemic circulation through the jugular vein, avoiding the liver “first-pass” effect. Data provided for chickens indicated that albendazole and fenbendazole are metabolized in avian species via the same metabolic pathway as in mammals (McKellar and Scott, 1990). As can be expected for dogs, cats, and man, the GI reductive metabolism of the BZD sulfoxide into the parent sulfides is markedly less relevant in poultry compared to ruminant species. Flubendazole is widely used as an anthelmintic in poultry and its main metabolites, hydrolyzed flubendazole and reduced flubendazole, have been identified in tissues of flubendazole-medicated turkeys. Understanding the role of the cuticular/tegumental structure on the process of drug uptake by helminth parasites is an area of major scientific interest. It has been shown from in vivo and ex vivo studies that transcuticular diffusion is the predominant pathway for the entry of anthelmintic drugs into nematodes (Cross et al., 1998; Alvarez et al., 2001). Although, there are relevant structural differences between the external surface of nematodes (cuticle) and cestodes/trematodes (tegument), the mechanism of drug entry to both types of helminths seems to be equally dependent on lipophilicity, as a major physicochemical determinant of drug capability to reach therapeutic concentrations within the target parasite. A high correlation between the octanol–water partition coefficients for different BZD anthelmintics and their capacity to diffuse through the tegument of Moniezia expansa has been shown (Mottier et al., 2003). The most lipophilic BZD compounds (fenbendazole, albendazole, mebendazole) (partition coefficients >3.7) were measured at higher concentrations within the tapeworm compared to those with the lowest lipid–water partition values (oxfendazole, albendazole sulfoxide, thiabendazole, etc.). A lower diffusion of albendazole and albendazole sulfoxide into Ascaris suum compared to Moniezia spp. and Fasciola hepatica has been demonstrated (Alvarez et al., 2001). Nematodes maintain a strongly buffered environment in the aqueous spaces of the cuticle structure, with a pH value of this compartment around 5.0 (accumulation of organic acids as products of carbohydrate metabolism). The lipid-containing region of the cuticle represents the rate-determining barrier for passive transport: the molecular size, restriction by pores, the intrinsic lipid–water partition coefficient, and pH/pKa relationship are among the rate-determining factors (Ho et al., 1992). BZD molecules largely exist in their ionized form at the acidic environment of the nematode surface, which may limit their diffusion across the cuticle. This ionization-mediated impairment of drug diffusion and the complex structure of the nematode cuticle compared to the cestode/trematode tegument, may explain the drug penetration differences observed between nematode and other helminths (Alvarez et al., 2001). In fact, this work demonstrated that the diffusion of BZD molecules into Fasciola hepatica and Moniezia spp. is markedly greater than that observed in the nematode Ascaris suum maintained under the same ex vivo conditions (Figure 39.8). Figure 39.8 Main routes of in vivo drug entry into different target helminth parasites. The identification of the capability of different helminth parasites to biotransform anthelmintic drugs is considered as another crucial step in the overall interpretation of the pharmacological activity of these drugs. It has been shown that both cytosolic and microsomal fractions obtained from different helminths are able to oxidized albendazole into its sulfoxide derivative. While this oxidative pathway is predominant in Fasciola hepatica, the sulforeduction of albendazole sulfoxide into its parent sulfide compound is the main metabolic activity identified in tapeworms (Solana et al., 2001). Additionally, the ex vivo biotransformation of albendazole to different albendazole glucoside metabolites was significantly higher in albendazole-resistant adult Haemonchus contortus compared to susceptible worms (Vokřál et al., 2013). The altered activities of certain detoxifying enzymes might partly protect the worms against the toxic effect of the drugs, contributing to a drug resistance mechanism. Although further investigation is required to establish the pharmacological relevance of drug biotransformation in target parasites, particularly regarding its potential impact on the development of resistance, these findings are complementary to studies addressing the understand of mechanisms of drug accumulation in helminths. Higher albendazole sulfoxide and oxfendazole compared to albendazole and fenbendazole concentrations have been reported in GI fluids of different ruminant species. However, the high lipophilicity of the parent thioethers (albendazole, fenbendazole) assures their penetration through the external surface of parasites. In fact, higher concentrations of albendazole were measured in Haemonchus contortus recovered from infected treated sheep compared to those of its sulfoxide metabolite (albendazole sulfoxide) (Alvarez et al., 2000). Since albendazole was not recovered in peripheral plasma, only drug from the pool found in abomasal fluid and mucosal tissue was available to reach the target nematode through its external cuticle. It is also likely that Haemonchus contortus may feed on portal blood. However, the low albendazole concentrations recovered in portal blood would not explain the large amount of albendazole recovered from this abomasal parasite. These findings may confirm the relevance of the transcuticular diffusion process, even in a blood-sucking parasite such as Haemonchus contortus. The higher anthelmintic potency of albendazole and fenbendazole and their greater capability to diffuse into the parasite, suggest that the parent drug may be the main molecule responsible for the activity against GI parasites. However, a complementary effect of the sulfoxide metabolites may contribute to the overall anthelmintic activity of these BZD compounds. On the other hand, transcuticular transfer appeared to be the main route of passage of other anthelmintic compounds, such as ivermectin, into the filarial nematode Onchocerca ochengi (Cross et al., 1998) and Haemonchus contortus (Lloberas et al., 2012). Drug concentration at the site of parasite location and the lipid solubility of the anthelmintic molecule are not the only parameters to consider when drug kinetics are evaluated. The physicochemical characteristics of the tissues and fluids surrounding the parasite may play a relevant role in drug accumulation. The partitioning of the active drug/metabolites between an aqueous gastrointestinal fluid and the lipoidal tissue of the parasite may facilitate the accumulation of the drug within the parasite. This drug partitioning phenomenon may be different for other sites of parasite location such as the biliary tract, where the bile-induced micelle formation may affect the diffusion of the active drug/metabolite into the target parasite (i.e., Fasciola hepatica). The physicochemical features of the environment where the target parasite is immersed play a pivotal role in the process of drug access, indicating that some helminths may be protected from the deleterious effect of an anthelmintic drug when living in their predilective location. This phenomenon may also explain many therapeutic failures observed in parasite control in both human and veterinary medicine, which in some cases have contributed to exposure of target parasites to subtherapeutic drug concentrations. BZD and pro-BZD were introduced into the animal health market primarily for the control of GI nematodes, not only for use in livestock animals (cattle, sheep, goats, swine, and poultry), but also for horses, dogs, and cats. The use of BZD and pro-BZD compounds quickly became widespread because they offered major advantages over previous available drugs in terms of spectrum, efficacy against immature stages and safety for the host animal. Additionally, BZD anthelmintics have ovicidal activity. With the exception of the halogenated BZD thiol, triclabendazole, which has only flukicidal activity against all stages of Fasciola hepatica (see Chapter 40), all BZD and pro-BZD can be classified as broad-spectrum anthelmintics. However, the substituted sulfur-containing methylcarbamates (albendazole, fenbendazole, etc.) and pro-BZDs (febantel, netobimin) have an extended spectrum of activity compared to the earlier BZD thiazolyls (i.e., thiabendazole). It has been demonstrated that the greater potency and spectrum of activity of the newer BZD is largely a function of their pharmacokinetic behavior rather than entirely due to their intrinsic differences in activity. Overall, the BZD methylcarbamate are broad-spectrum anthelmintics active against a variety of GI and lung nematodes, tapeworms, and trematodes (albendazole derivatives). As a consequence of their pharmacokinetic behavior in monogastric species (particularly in dogs and cats), the BZDs are more effective when they are administered for several days, compared single-dose treatments. The available commercial formulations and indications for use (route of administration, dosage, and target animal species), may vary among different countries. Readers interested on any specific issue on the spectrum of activity for BZD compounds are referred to previous editions of this textbook (see Reinemeyer and Courtney, 2001). Some general considerations on the spectrum of activity for the most widely used BZD and pro-BZD compounds are summarized below. Thiabendazole is indicated for the treatment and control of GI roundworms in horses, cattle, sheep, and goats, and the control of lungworms in sheep. Thiabendazole is administered orally at the dose range 66 to 110 mg/kg. It is also used as a fungicide for crop protection. In horses, thiabendazole is used as a single oral dose administered as a drench or by stomach tube, or as an oral paste. It is usually associated with piperazine to increase the efficacy against ascarids and immature Oxyurus spp. Thiabendazole is ineffective against cestodes or trematodes. In cattle, at the recommended dose rates, it is effective against adult and developing stages of Ostertagi ostertagi but not against stage EL4. Thus, thiabendazole is not useful to prevent type II ostertagiasis. In pigs, thiabendazole has little efficacy against Ascaris suum and Trichuris suis. The pro-BZD netobimin is formulated as a suspension for oral administration in cattle and sheep. It is indicated (7.5 mg/kg) for the removal and control of tapeworms (heads and segments)(Moniezia expansa, M. benedeni), adults and larval stages of abomasal worms (Haemonchus contortus, H. placei, Trichostrongylus axei, Ostertagia ostertagi, Teladorsagia circumcincta, Marshallagia marshalli), intestinal worms (Trichostrongylus colubriformis, Chabertia ovina, Nematodirus spathiger, N. filicollis, N. helvetianus, Cooperia oncophora, C. punctata, Bunostomun phlebotomum, Oesophagostomum radiatum), and lungworms (Dictyocaulus filaria, D. viviparus). Higher doses (20 mg/kg) are required for control of adult liver flukes (>14 weeks old) (Fasciola hepatica, Fascioloides magna) and Thysanosoma actinioides, and type II ostertagiasis (cattle). Albendazole is formulated as a suspension for oral administration (as a drench) in cattle and sheep at the recommended dose of 7.5 and 5 mg/kg, respectively. It is indicated for the removal and control of a broad spectrum of helminth parasites, including: tapeworms (heads and segments), abomasal and intestinal nematodes (adults and fourth-stage larvae), and lungworms (adults and larval stages). Additionally, at 10 mg/kg (cattle) and 7.5 mg/kg (sheep), albendazole is active against adult liver flukes (>14 weeks old). Ovicidal activity has also been demonstrated at 8 hours posttreatment. Albendazole appeared to be effective in suppressing cyst excretion by Giardia-infected calves, at the oral dose of 20 mg/kg once daily for 3 days; it is thus a potential antigiardial drug for use in farm animals (Xiao et al., 1996). Similar results were observed in Giardia-infected dogs after oral administration (25 mg/kg) twice a day for 2 days (Barr et al., 1993). Ricobendazole is the sulfoxide metabolite of albendazole and it is also available as a suspension for oral administration in cattle (10 mg/kg) and sheep (7.5 mg/kg). The spectrum of activity is equivalent to that described for albendazole, including the ovicidal effect. A novel ricobendazole injectable solution (15%) for subcutaneous administration to cattle is available in some countries. It has been successfully used to control abomasal and intestinal nematodes and lungworms. A 5 mg/kg dose rate is recommended to control the larval fourth stage of O. ostertagi. The formulation is not recommended for control of liver flukes and tapeworms. In Europe, ricobendazole is also approved for use in pheasants by feed administration (17 mg/kg during 3 days) and is mainly indicated for the control of ascarids and capillarid infections. Febantel, a pro-BZD compound, is used in ruminants, horses, dogs, cats, and pigs. It is formulated as a paste, suspension, or tablets for oral administration. It is used at a dose of 10 mg/kg (ruminants), 6 mg/kg (horses), 10 mg/kg (dogs and cats) and 20 mg/kg (pigs). Dogs and cats required a 3-day period of treatment. Since FBT has not anthelmintic activity, its spectrum depends on its main active metabolites fenbendazole and oxfendazole. Consequently, its spectrum is similar to these compounds. Fenbendazole is formulated as a suspension for oral administration in cattle, sheep, and goats at the recommended dose of 10 mg/kg (cattle) and 5 mg/kg (sheep, goats). Fenbendazole is indicated for the removal and control of tapeworms (heads and segments), abomasal and intestinal nematodes (adults and fourth stage larvae), and lungworms (adults and larval stages). Fenbendazole also have ovicidal activity. Fenbendazole is effective for the treatment of Giardia infections in calves at an oral dose of 10 mg/kg administered once, or 5 mg/kg given every 24 hours for a 3-day period (O’Handley et al., 1997). Fenbendazole is administered orally to horses at 5 mg/kg for control of large strongyles (Strongylus vulgaris, S. edentatus, S. equinus), small strongyles (cyathostomes), and pinworms (Oxyuris equi). Higher doses (10 mg/kg) are required for ascarids (Parascaris equorum) control. At 10 mg/kg for 5 consecutive days, fenbendazole is indicated for control of arteritis caused by the fourth stage larvae of S. vulgaris, encysted mucosal cyathostomes larvae, including early (hypobiotic) and late third stages larvae. In pigs, fenbendazole is used as a powder (3 mg/kg per day for 3 consecutive days), and is 99% effective against Ascaris suum larvae migrating through the liver and lungs, and most GI nematodes (mature and immature) (Metastrongylus apri, Oesophagostomun dentatum, O. quadrispinulatum, Hyostrongylus rubidus, Trichuris suis, Stephanurus dentatus). Fenbendazole is used at 50 mg/kg daily for 3 consecutive days in dogs for removal of hookworms (Ancylostoma caninum), ascarids (Toxocara canis, Toxascaris leonina), whipworms (Trichuris vulpis), and tapeworms (Taenia). However, is not recommended for control of the cestode Echinoccocus granulosus or Echinococcus multilocularis. A 50 mg/kg dose for 3 days achieves good therapeutic effect against Giardia in dogs (Barr et al., 1993). The use of fenbendazole (granules) in zoo and wildlife animals has been approved by the Food and Drug Administration (USA). It is used at 10 mg/kg during 3 days, orally administered with food, and is indicated for the control of nematodes (ascarids, hookworms, and tapeworms) of Felinae (lion, tiger, puma, cheetah, leopard) and Ursidae (black bear, polar bear). Oxfendazole is administered orally to cattle (4.5 mg/kg), sheep (5 mg/kg), and horses (10 mg/kg). Additionally, has been used in goats, dogs, and pigs. In all cases it is recommended for the control of the same parasites as its sulfide parent compound (fenbendazole). To avoid the ruminal bypass, an oxfendazole suspension has been approved for intraruminal administration in cattle. As described for albendazole and fenbendazole, oxfendazole can be used for the prevention and control of both type I and type II ostertagiasis in cattle. However, variable efficacy against stage EL4 has been reported. A probable explanation for the observed variability in efficacy is the metabolic activity associated to the larval stage, where the highest efficacy could be expected during induction of inhibition and emergence of inhibited larvae and the lowest efficacy during the quiescent period. In horses, oxfendazole have a similar efficacy against GI nematodes, like the parent fenbendazole. Oxfendazole has been demonstrated to have activity against Taenia solium cysticercus in pigs after a single oral administration at 30 mg/kg (Gonzales et al., 1996), which has been proposed as a tool to interrupt the transmission cycle of this parasite, protecting people from neurocysticercosis. Additionally, in controlled efficacy tests using naturally infected pigs, this dosage regimen has been demonstrated to be effective against adult liver flukes (Ortiz et al., 2014) and adult stages of Ascaris suum, Oesophagostomum spp., T. suis, and Metastrongylus spp. (Alvarez et al., 2013). Oxibendazole is used in the treatment of adult and larval stages of GI nematodes of pigs, cattle, sheep, and horses. It is used orally in suspension or paste at a dosage of 10 mg/kg (sheep and cattle) or 15 mg/kg (horses). A 10 mg/kg dose in horses is sufficient for removal and control of large strongyles, small strongyles, large roundworms, and pinworms. Mebendazole is administered orally to horses (8.8 mg/kg), sheep, and goats (15 mg/kg). It is also used in game birds, pigs, deer, dogs, and poultry as different types of formulations: premixes for medicated feed, pastes, tablets, granules, and drenches, all for oral administration. It is indicated for the control of large strongyles, small strongyles, large roundworms, and mature and immature larval stages of pinworms in horses. Doses of 22 mg/kg for 3 consecutive days are recommended for use in dogs for the treatment of roundworms, hookworms, whipworms, and tapeworms. Flubendazole is available as tablets, pastes, pellets, and premixes for oral administration in pigs, chickens, turkeys, and game birds. It is used at 5 mg/kg (single oral administration) and at 30 mg/kg in feed for 10 days in pigs, and is active against lungworms, roundworms, nodular worms, and whipworms. A 10-day feed period is required for control of heavy Trichuris suis infections. Oral treatment at 22 mg/kg for 3 consecutive days is recommended in dogs and cats for removal of common GI parasites and tapeworms. Flubendazole is used in poultry at 60 mg/kg in feed for 7 days (equivalent to 5 mg/kg/day for broilers and 3.6 mg/kg/day for laying hens). Flubendazole has ovicidal activity and work done in sheep demonstrates that flubendazole has a volume of distribution higher than those reported for albendazole and oxfendazole (Moreno et al., 2004), which may offer a new attractive alternative for use of the drug against tissue-dwelling parasites in sheep, where the presence of high drug concentrations for an extended period of time is usually crucial to achieve optimal clinical efficacy. As discussed in Section Pharmacokinetic Behavior, BZD anthelmintic compounds are virtually insoluble in water, which limits most of the formulations to suspension, paste, granule, tablets, blocks, powder, and pellets for oral or intraruminal administration, or for administration in feed. Drench formulations are most frequently used in ruminant species, pastes are often preferred for horses, tablets for dogs and cats, and feed administration (powder) for swine and poultry. BZD are generally administered to ruminants in the form of a single oral dose. This single-dose treatment has traditionally been done by oral drench or by intraruminal injection; this latter approach is based on the design of a special syringe (intraruminal injector) that ejects the drug directly into the rumen cavity. This device is commercially available for the administration of a concentrated suspension of oxfendazole to cattle and was mainly designed to overcome the potential problems of the esophageal groove closure after oral treatment (see Section Factors Affecting the Disposition Kinetics and Efficacy of Benzimidazole Anthelmintics). To reduce the cost associated with treating large number of animals and with growing evidence that divided anthelmintic doses and prolonged administration increase anthelmintic efficacy, several methods for drug delivery have been used in the past. The incorporation of drug into feed blocks, for ingestion of small amounts over a prolonged grazing period, and the inclusion of drugs in drinking water, have been used for therapeutic and prophylactic parasite control. Convenience and labor saving are obvious with these group-medication systems, but at the same time no direct control is possible over the drug intake rate of individual animals. The most versatile technology in anthelmintic drug delivery has been the development of ruminal devices that, when given to individual animals, can deliver drugs for an extended period of time. These controlled-release devices or boluses more readily provide the conditions for increased anthelmintic efficacy, such as prolonged exposure of parasites to sustained concentrations of active parent drugs or their metabolites. However, due to the high selection pressure, this sustained exposure may facilitate the development of drug-resistance parasite populations. After oral administration, the boluses remain in the rumen-reticulum and release the drug over a long period of time, either in a sustained or pulsatile manner. Different controlled-release systems have been developed for the delivery of BZD anthelmintics. The oxfendazole pulse release bolus (Rowlands et al., 1988) is based on principle of releasing a series of individual therapeutic doses of oxfendazole at predetermined intervals (20–21 days), which approximately coincide with the prepatent period of the major parasites nematodes of cattle, for about 4 months. This is achieved by the continuous galvanic corrosion of a magnesium alloy rod that periodically exposes an annular oxfendazole tablet to the ruminal fluid (Campbell, 1990). An intraruminal slow-release capsule which delivers, in the rumen of sheep and cattle, a low daily dose of albendazole for approximately 3 months has been designed. The device is a hollow cylinder of nonbiodegradable plastic which contains tablets of 3.85 g (sheep) and 18.46 g (cattle) of albendazole, and two external wings. After the administration, the wings spread out while the ruminal fluid starts to dissolve the first tablet, which releases the drug slowly in the rumen; a metal spring pushes six tablets toward the open end of the capsule. Following the administration of this device, albendazole metabolites were found in plasma for 90 days (cattle) and 105 days (sheep) after capsule administration (Delatour et al., 1990b). Albendazole is also available in controlled-release capsules, which contain 2.1 and 3.85 g of albendazole/capsule for use in weaner and adult sheep, respectively, showing a sustained anthelmintic action for 90 to 100 days after administration. Additionally, an ivermectin/albendazole capsule has been developed to assure protection against GI parasites susceptible to either compound (Castells et al., 2011). Several host-related factors may affect the kinetic behavior and resultant clinical efficacy of BZD compounds. Dissolution, absorption, and biotransformation are three of the most important processes affected by a series of host-related factors. Consult Chapter 4 in this text for background to these phenomena. Manipulation of the pharmacokinetic and metabolic patterns and the comprehension of different factors modulating them seem to be excellent alternatives to improve the use of BZD in ruminants.
Antinematodal Drugs
Benzimidazoles and Probenzimidazoles
Chemistry
Pharmacodynamics: Mode of Action
Pharmacokinetic Behavior
Pharmacokinetics and Metabolism in Ruminant Species
Absorption
(1) Route of administration: only oral or intraruminal routes can be used for the sulfide compounds (ABZ, FBZ).
(2) Rumen: marked drug dilution. The rumen’s reservoir effect is by drug adsorption to particulate digesta.
(3) Abomasum: the acidic pH facilitates drug particles dissolution and strong “ionic trapping” of the drug/metabolite at the abomasum.
(4) Small intestine: main drug absorption site.
(5) Liver: drug oxidative metabolism.
(6) Biliary system: drug excretion (unconjugated and conjugated metabolites), enterohepatic recycling.
(7) Bloodstream: metabolites distribution to different organs/tissues, including active secretion to gastrointestinal tract.
(8) Rumen: sulforeductive biotransformation by ruminal microflora.
ABZ, albendazole parent compound; ABZSO, albendazole sulfoxide; ABZSO2, albendazole sulfone.
Metabolism and Elimination
Hepatic and extrahepatic oxidative metabolism:
Reductive Metabolism in the Gastrointestinal Tract
Distribution to Parasite Location Tissues
Pharmacokinetics in nonruminant species
Mechanisms of Drug Transfer into Target Parasites
Anthelmintic Spectrum
Thiabendazole
Netobimin
Albendazole
Ricobendazole
Febantel
Fenbendazole
Oxfendazole
Oxibendazole
Mebendazole
Flubendazole
Routes of Administration and Drug Formulations
Factors Affecting the Disposition Kinetics and Efficacy of Benzimidazole Anthelmintics
Effect of ruminant esophageal groove closure:
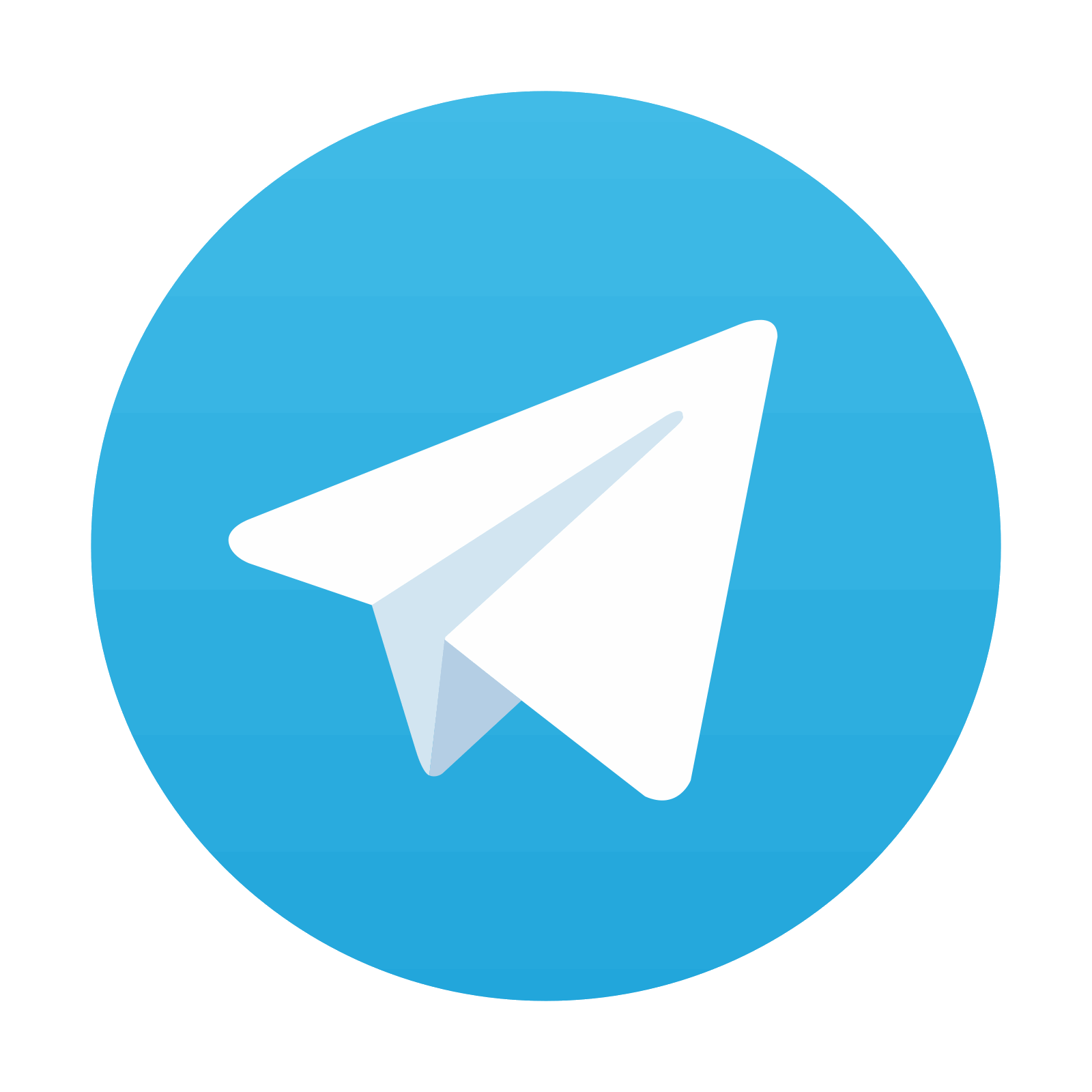
Stay updated, free articles. Join our Telegram channel
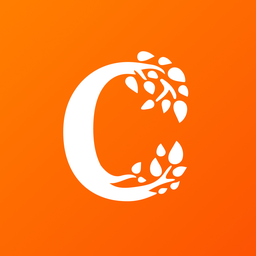
Full access? Get Clinical Tree
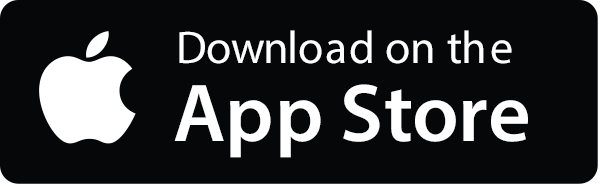
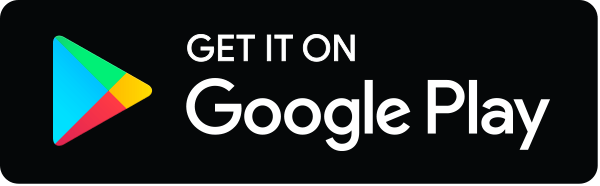