and Mary C. Olmstead2
(1)
Medicine Hat College, Medicine Hat, AL, Canada
(2)
Department of Psychology, Queen’s University, Kingston, ON, Canada
Abstract
Eating disorders and drug addiction share many common traits. This includes biological and environmental factors that predispose individuals to develop either disorder, an increased risk for anxiety and depression when the disorders are present, and heightened trait levels of impulsivity and compulsion. Animal models of eating disorders are not as well established as those that model drug addiction, but the research in this area is progressing rapidly. In this chapter, we discuss anorexia nervosa, bulimia nervosa, binge eating disorder, and obesity as these encompass the majority of maladaptive eating behaviors in humans. We begin by outlining the important features that characterize each disorder and that should thereby be present in an animal model. An overview of peptide control of feeding is provided to help the reader evaluate the animal models presented. These are based principally on genetic variation and stressful life events. In general, most animal models based on genetic alterations have limited applicability to humans, at least to date. Those based on stressful life events appear more promising in that they more accurately reproduce alterations in feeding and neuroendocrine function that are characteristic of each disorder. The next obvious step in eating disorder research is to combine the two approaches to determine how genetic alterations and stressful events interact to produce maladaptive eating and physiological changes.
Key words
Anorexia nervosaBulimia nervosaBinge eating disorderObesityStressGeneticsNeuroendocrine functionFeeding peptidesHypothalamus1 Introduction
Eating disorders and drug addiction share many common traits. This includes biological and environmental factors that predispose individuals to develop either disorder, an increased risk for anxiety and depression when the disorders are present, and heightened trait levels of impulsivity and compulsion. Like drug addiction, the chance of recovery from eating disorders is dismally low: more than 50% of patients in partial remission relapse within a year (1, 2). The overlap between eating disorders and drug addiction should not be surprising as drugs of abuse activate neural systems that evolved to reinforce behaviors, such as feeding, that enhance species’ survival (3, 4).
Animal models of eating disorders are not as well established as those that model drug addiction, but the research in this area is progressing rapidly. As with drug addiction, no single paradigm can model the complexity of pathological eating, particularly as this is a large category that can be divided into several distinct disorders. In this chapter, we discuss anorexia nervosa, bulimia nervosa, binge eating disorder, and obesity as these encompass the majority of maladaptive eating behaviors in humans. Anorexia nervosa is characterized by self-imposed weight loss to a body weight less than 85% of that expected for the individual’s age and height. Anorexics exhibit increased activity levels and food obsessions (related to the handling and preparing of food), but no significant disturbances in appetite (5). The disorder may be separated into subtypes: restricting and binge eating/purging. Bulimia nervosa is sometimes considered a subtype of anorexia nervosa as both involve excessive preoccupation with food and body image, and bulimia is often preceded by a history of anorexia. The defining feature of bulimia is repeated episodes of overeating followed by compensatory behaviors such as vomiting, laxative use, or excessive exercise. Thus, the binge eating/purging subtype of anorexia nervosa overlaps considerably with bulimia nervosa, with the exception that anorexics are underweight whereas bulimics are typically of normal to low weight. Recurrent bouts of extreme overeating also occur in binge eating disorder, but with no subsequent compensatory behavior. Obesity is distinct from these other three disorders in that maladaptive eating (either restricted or uncontrolled) is not a criterion for this condition: obesity is defined solely as a state of excess body fat with a corresponding body mass index (BMI) greater than 30 (6). Indeed, obesity is not even listed as a psychiatric disorder in DSM-IV (7). Nonetheless, with very few exceptions, obese individuals display disrupted eating patterns and/or activity levels that jeopardize their health. By this criterion, obesity is clearly an eating disorder and is discussed in this chapter as such. Moreover, the increasing prevalence of obesity makes it a global health issue and one that requires the attention of the research community.
Given that a primary feature of all eating disorders is disrupted feeding, any animal model must reproduce the maladaptive eating that is characteristic of that disorder. In the case of anorexia nervosa, food restriction should be self-imposed such that animals forego food consumption even when it is readily available. Despite the reduced intake of calories, anorexics exhibit normal to high activity levels, which should also be manifested in an animal model. Bulimia nervosa cannot be modeled fully in animals as there is no evidence that rodents or nonhuman primates initiate compensatory behaviors following binge eating episodes. By that criterion, gut emptying via gastric fistula does not model purging. In contrast, binge eating can be modeled effectively in animals if brief bouts of excessive food intake occur repeatedly over time, are enhanced by food palatability, but are independent of food deprivation and circadian rhythms. To conform to the human disorder, food consumption of bingeing animals must be exaggerated compared to control animals exposed to the same environmental conditions. Obesity can be reproduced in animals through a variety of means, but the relationship between this state and the human condition depends on whether these animals also exhibit alterations in food intake. We use this as a criterion to evaluate animal models of obesity because, with the exception of a very small percentage of people, obese humans show different patterns of food consumption than do individuals of normal weight.
In addition to these fundamental changes in feeding behavior, physiological and neuroendocrine changes accompany most eating disorders. A detailed analysis of these changes in anorexia nervosa suggests that some, but not all, are adaptations to reduced food intake and weight loss (8). Bulimics, binge eaters, and obese humans exhibit distinct profiles of neuroendocrine changes although, in the absence of large longitudinal studies, it is impossible to determine which predates the other. Regardless of the causal relationship, the same changes should be evident in animal models of a particular disorder. Finally, any animal model should attempt to explain the higher rates of all eating disorders in females and, with the exception of obesity, the increased propensity for adolescent onset. In the following sections, we evaluate animal models of eating disorders, based on how well they reproduce altered eating patterns and the accompanying physiological and neuroendocrine changes characteristic of each disorder.
2 Peptide Control of Feeding
In order to evaluate animal models of eating disorders, it is important to understand the complex network of central and peripheral signaling peptides that control feeding, simplified in Fig. 1. Food intake and body weight are regulated, primarily, through neuropeptide production in the hypothalamus. The arcuate nucleus in this structure contains two groups of interconnected, “first-order” neurons that send axon terminals into the hypothalamic median eminence. The blood brain barrier is incomplete in the median eminence, allowing these neurons to contact the bloodstream (9). One group of first-order neurons produces anorexigenic (appetite-suppressing) peptides such as cocaine- and amphetamine-regulated transcript (CART) and the prohormone pro-opiomelanocortin (POMC). Cleavage products of POMC include the melanocortins, adrenocorticotropic hormone (ACTH), and alpha-melanocyte-stimulating hormone (α-MSH), the latter of which acts as an agonist at melanocortin-3 (MC3) and -4 (MC4) receptors to suppress food intake and weight gain (10). MC3 receptors are located primarily within the arcuate nucleus whereas MC4 receptors are found in many hypothalamic nuclei and widely distributed throughout the brain (11, 12). The second group of first-order neurons acts in opposition to the first, releasing orexigenic (appetite-stimulating) peptides that include neuropeptide Y (NPY) and agouti-gene-related protein (AgRP). AgRP acts as an endogenous antagonist at MC3 and MC4 receptors (10, 11), helping to maintain a balance between signals that increase and decrease food intake.
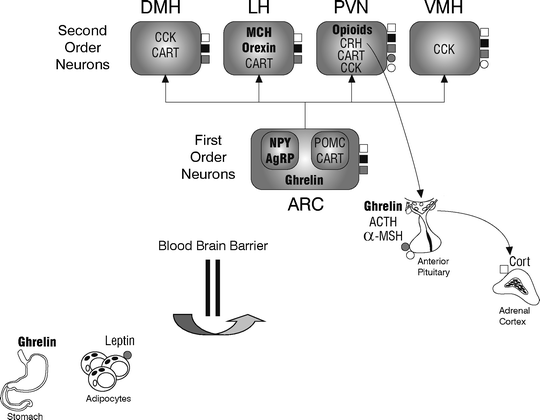
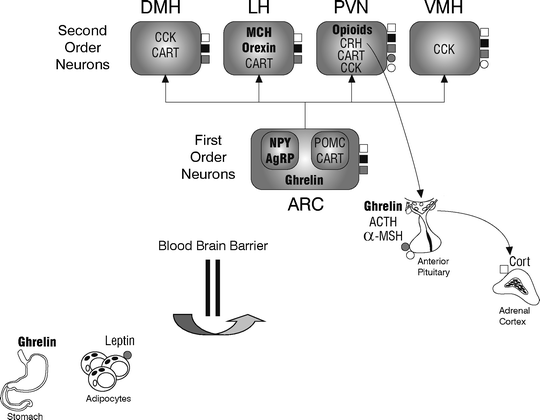
Fig. 1.
Schematic representation of peptidergic control of feeding. Information about the body’s energy balance originates in peripheral organs, including the stomach and adipose tissue. Peptides, such as ghrelin and leptin, cross the blood brain barrier to convey signals to first-order neurons in the arcuate nucleus (ARC) of the hypothalamus. These project to second-order neurons in the dorsomedial nucleus (DMH), lateral nucleus (LH), paraventricular nucleus (PVN), and ventromedial nucleus (VMH) of the hypothalamus. Feeding-related neuropeptides within each hypothalamic nucleus are represented by thick (orexigenic) and thin (anorexigenic) fonts. ❑ melanocortin (MC) receptors; ■ leptin receptors; □ ghrelin receptors; ❍ CRH receptors; ● glucocorticoid receptors. Refer to the text for information on neuropeptide function and interactions with the stress hormone system.
First-order neurons in the arcuate nucleus send signals to “second-order” neurons in the dorsomedial, ventromedial, lateral/perifornical, and paraventricular nuclei of the hypothalamus. Within second-order nuclei, orexigenic and anorexigenic peptides are co-localized and co-released, activating receptors in overlapping regions. For instance, the lateral/perifornical hypothalamus contains the orexigenic peptides melanin-concentrating hormone (MCH) and orexin, as well as the anorexigenic peptide, CART. The paraventricular nucleus contains the anorexins cholecystokinin (CCK) and corticotropin-releasing hormone (CRH), but also orexigenic endogenous opioids. Whether orexigenic or anorexigenic peptides are released is largely dependent upon peripheral signals relating to the body’s energy balance. One such signal comes from leptin, an anorexigenic peptide produced by adipocytes (fat cells) in direct relation to fat tissue mass. Leptin activates Ob receptors, the product of the diabetes (Db) gene (13), within the median eminence; it also crosses the blood brain barrier to activate Ob receptors located on orexigenic- and anorexigenic-producing neurons in the arcuate, dorsomedial, ventromedial, lateral/perifornical, and paraventricular nuclei. In a state of positive energy balance, this adiposity signal reaches the arcuate nucleus where POMC and CART neurons are stimulated and NPY and AgRP neurons are inhibited. In a negative energy balance, the opposite occurs. Ghrelin is another peripheral signaling peptide, produced by the stomach and, in smaller amounts, by the arcuate nucleus and pituitary (14, 15). In contrast to leptin, ghrelin exerts orexigenic effects by stimulating NPY and AgRP expression in the hypothalamus (16, 17) and by antagonizing the leptin pathway (18). It is through these mechanisms that peripheral signals play an important role in regulating hypothalamic feeding-peptide function.
3 Animal Models Based on Genetic Variation
Anorexia nervosa, bulimia nervosa, binge eating disorder, and obesity are all associated with genetic variations in the peptide feeding system described above. This may explain, at least partially, differences in the vulnerability to develop these disorders. Indeed, estimates for the heritability of anorexia range from 33% to 84%, bulimia from 28% to 83%, and binge eating disorder from 38% to 61% with the remaining variance dependent upon individual experiences (19, 20). Heritability of obesity is the most substantial of all eating disorders, ranging from 77% to 94%. It is not surprising, therefore, that the majority of animal models based on genetic variation relate to this disorder.
3.1 Obesity
The human obesity gene map (2005 update) references 135 candidate genes and more than 600 loci where mutation or transgenic expression in mice alter body weight and adiposity (21). Heritability of obesity, therefore, is probably polygenetic and likely interacts with environmental factors such as over-responsiveness to external stimuli (22) or the availability of calorically dense comfort foods (3). Despite the difficulty of establishing gene–obesity connections, animal models of spontaneous single-gene mutations have provided valuable insight into the neuroendocrine changes that accompany obesity. Currently, there are 10 spontaneous single-gene mutations in mice and rats that result in an obese phenotype (23); those that are relevant to human obesity (24) are discussed below.
The most common genetic alterations that produce obesity in animals involve dysfunction in leptin signaling. Early experiments indicated that the profoundly obese ob/ob mouse lacks a circulating factor (25), later identified as leptin (26, 27). The animals exhibit hyperphagia combined with reduced locomotor activity and metabolic rate (28, 29). Importantly, this phenotype is normalized by exogenous leptin administration (29, 30). Loss-of-function mutations in the leptin receptor gene result in a similar phenotype, as seen in the diabetic db/db mouse and the Zucker fatty fa/fa rat (31). Deletion of hypothalamic Ob receptors does not produce profound obesity characteristic of ob/ob mice, although the reduction in central leptin receptors correlates with the severity of obesity in these animals (32, 33). The leptin-induced control of food intake appears to be mediated through interactions with hypothalamic peptides: obese and leptin-receptor deficient rodents show decreased POMC and CART expression, increased AgRP, NPY, and melanin-concentrating hormone expression, and are less sensitive to CCK-induced reductions in food intake and meal termination (31, 34–40).
Like animals, humans may exhibit genetic deficiencies in leptin production and loss-of-function leptin-receptor mutations. These alterations produce hyperphagia, severe early-onset obesity, and rapid weight gain during development (41). In leptin-deficient individuals, leptin administration normalizes hyperphagia and dramatically reduces body weight and adiposity. Congenital leptin and leptin-receptor deficiencies are extremely rare and cannot explain the growing obesity epidemic. On the other hand, serum leptin and adipocyte Ob gene expression are strongly correlated with percent body fat in obese individuals. This suggests that, even in the absence of congenital leptin-signaling defects, obesity may represent a self-propelling state wherein high serum leptin levels lead to resistance and a loss of leptin-induced appetite suppression (42). The proposed mechanisms of leptin resistance include deficiencies in the leptin blood-brain-barrier transport system (43, 44), impaired rhythmicity of plasma leptin levels (45), adipocyte leptin-receptor blockade (46), and leptin signaling defects within the hypothalamus (47). Leptin resistance may be regulated, at least partially, by external factors as mice show increased resistance to leptin during consumption of a high-fat diet (48).
Hyperphagia, obesity, and increased linear growth rate also occur with genetic alteration of the melanocortin system, which includes POMC, α-MSH, AgRP, and MC4 receptors. POMC gene deletion in the mouse creates a loss of α-MSH signaling at MC4 receptors, resulting in hyperphagia and obesity that can be partly reversed by peripheral α-MSH treatment (49). Human POMC gene mutations have been linked, as in the animal model, to overeating and early-onset obesity (50). These mutations need not be homozygous: individuals exhibiting heterozygous point mutations that reduce hypothalamic MC4 receptor signaling have a high prevalence of obesity, suggesting that even partial defects in melanocortin function predispose individuals to obesity (51). Further, genetic deficiencies in the production of POMC cleavage enzymes, such as prohormone convertase 1, result in severe early-onset obesity (52). Like POMC gene deletion, targeted deletion of the MC4 receptor in mice produces hyperphagia and obesity (53, 54). MC4 receptor knockout mice are particularly susceptible to increased weight gain on a high-fat diet (55). These findings accord with evidence that the MC4 receptor selectively modulates dietary fat intake, as MC4 receptor knockout mice do not exhibit hyperphagia when placed on a low-fat diet, and MC4 receptor agonists selectively reduce fat, but not protein or carbohydrate, consumption (56). Like leptin-deficient animals, MC4 receptor knockout mice are largely insensitive to CCK-induced satiety (57).
Consistent with animal studies, MC4 receptor gene mutations in humans contribute to hyperphagia, increased fat mass, and increased growth rate in 0.5% to 1.0% of obese adults and 6% of individuals with childhood-onset obesity (24, 58–63). Although homozygous mutations produce a more severe phenotype, heterozygous mutations and the resultant loss of MC4 receptor function also lead to hyperphagia and obesity (59). In addition, DNA sequence variation in the regulatory region of the MC4 receptor gene is associated with increased waist circumference and fat mass (64, 65). One MC4 receptor variant, discovered in 25% of severely obese individuals, corresponds with increased intake of high-fat and high-protein foods (66). Overexpression of the AgRP gene decreases MC4 receptor activity and increases obesity in mice (67), and obese humans exhibit high levels of plasma AgRP (68). In rats, intracerebroventricular injections of AgRP selectively enhance intake of high-fat, but not low-fat, foods (69), reminiscent of the human MC4 receptor-variant phenotype (66). Genetic variation in the human AgRP gene (70) as well as an upstream promoter region (71) are associated with late-onset obesity. These animal models of genetic variation highlight the importance of heritability in susceptibility to obesity. They also demonstrate that neuropeptide feeding system function is modified by environmental factors, such as high-fat diet consumption, as these may influence gene expression and other processes that maintain balance in the feeding peptide system.
3.2 Anorexia Nervosa, Bulimia Nervosa, and Binge Eating Disorder
Like obesity, anorexia nervosa is strongly familial (72), and is associated with genetic mutation or variation. Still, there is considerable debate regarding susceptible genes and this disorder (73). The most widely implicated candidate genes for anorexia are those encoding serotonin (5-HT) receptors, promoter regions, and transporter proteins (73–79). The anx/anx mouse supports this proposed connection between disruptions in 5-HT function and anorexia. This spontaneous mutation produces increased 5-HT neurotransmission (80, 81) and anorexic traits early in life, including dysregulated food intake, failure to grow, emaciation, and hyperactivity (82). The anx/anx mouse also exhibits reduced serum leptin levels and abnormalities in hypothalamic neuropeptide systems, including NPY, AgRP, POMC, and CART (83, 84), changes that are associated with anorexia in humans. These animals, however, also display traits uncharacteristic of the disorder, including body tremors, ataxia, and head weaving (82). Many of the animals die between 20 and 30 days of age, severely restricting the validity of this model.
Other proposed animal models of anorexia also lack validity to the human condition. This includes models based on inactivation of genes encoding melanin-concentrating hormone (85), CRH type 2 receptors (86), muscarinic M3 receptors (87), and tyrosine hydroxylase (88). Although all of these models replicate some feature of anorexia (e.g., hypophagia, emaciation, hypoleptinemia, altered metabolic rate, and/or neuropeptide system dysfunction), genetic studies in humans have failed to support their validity (79). Similarly, a number of neuropeptide systems are dysregulated in anorexia nervosa (8, 89), but there is little evidence that these are candidates for genetic predisposition to the disorder (73). The development of an animal model based on these irregularities, therefore, is unwarranted. Variations in the delta-1 opioid receptor gene are associated with anorexia in humans (74, 75, 90), but an animal model based on this variant has yet to be developed. Finally, binge/purge anorexia (91), bulimia (92, 93), and binge eating disorder (94) have all been linked to variants of ghrelin or preproghrelin genes, but these findings are not universal (95, 96) and ghrelin knockout mice show no alterations in 24-h food intake or body weight gain (97).
In contrast to these poorly validated models, there are a handful of animal models based on genetic variation that show promise for understanding eating disorders. First, variation in the DNA sequence encoding the AgRP gene is associated with the restricting subtype of anorexia nervosa (98) and central AgRP infusions increase food intake and prevent starvation in a rat model of anorexia (99). Second, restricting and binge/purge subtypes of anorexia have been associated with different alleles of the central cannabinoid receptor 1 (CB1) gene (100), and CB1 receptor knockout mice eat less than wild-type mice following temporary food restriction (101). Third, both anorexia and bulimia nervosa are linked to variants of genes encoding estrogen receptors (102–104), which may account, in part, for the increased prevalence of these disorders in females (105). In rats, estrogen increases activity of the CCK signaling pathway and reduces food intake and body weight (106, 107). Fourth, hypothalamic MC4 receptor signaling modulates meal size and meal choice (108), and early-onset obesity in MC4 receptor knockout mice is the result of hyperphagia (109). Moreover, in patients undergoing laparoscopic gastric banding as a treatment for obesity, 6.3% carried MC4 receptor gene variants and all met the criteria for binge eating disorder (110). Similarly, in a sample of severely obese individuals, 5.1% exhibited MC4 receptor mutations with 100% of carriers reporting binge eating; in obese and normal-weight subjects without MC4 receptor mutation, rates of binge eating were 14.2 and 0%, respectively (111). MC4 receptor mutation is not, however, always associated with an increased risk for binge eating (112, 113), emphasizing that other factors play an important role in the etiology of this disorder.
Many animal models of psychiatric disorders, including some discussed above, produce genetic alterations using gene knockout technology. These studies must be interpreted with caution because the redundancy of orexigenic pathways allows ample opportunity for compensation of function during development. This may explain some apparent discrepancies, such as the finding that NPY modulates eating, but NPY gene deletion does not affect food intake or body weight (114). It should also be noted that our preceding discussion focused on genetic alterations related to the neuropeptide feeding system. In contrast, eating disorders are complex psychiatric conditions that undoubtedly involve disturbances in a variety of cognitive and behavioral processes. Both animals and humans that exhibit these changes may display genetic alterations in neural systems that mediate reward, emotion, learning, or inhibition, among others.
4 Animal Models Based on Stressful Events
Eating disorder patients exhibit heightened responses to stress, particularly as a trigger for binge eating (115). Obese individuals are stress-sensitive in that overeating in these individuals is more likely to be triggered by internal stress responses than by physiological signals of hunger. It is not surprising, therefore, that stressful life events often precipitate anorexic, binge eating, or bulimic episodes (8, 106, 116–118) which, over time, may lead to obesity (119).
The effect of stress on food intake is moderated by stressor intensity in both humans and animals: mild or acute stress increases eating whereas severe stress decreases eating (120–122). When foods high in fat and sugar are available, stress increases intake of these “comfort” foods (119, 123), even under conditions when reductions in eating are normally exhibited (124). Given the well-established links between stress and eating disorders (115), a number of animal models have been developed that show altered food consumption and body weight following stress. Prior to discussing these, we briefly outline interactions between stress hormones and feeding peptides, providing a framework for discussing animal models based on stress responses.
4.1 Stress Hormone- Feeding Peptide Interactions
Stress, defined as a physical or psychological event that causes a disruption in homeostasis (125), activates the hypothalamic–pituitary–adrenal (HPA) axis initiating a cascade-like secretion of hormones. The stress response is initiated in the paraventricular nucleus with the release of CRH. CRH stimulates high-affinity type 1 (CRH1) receptors located on anterior pituitary corticotrophs (126), resulting in ACTH and α-MSH secretion as well as increased POMC synthesis and gene transcription (127). Although ACTH and α-MSH suppress food intake, the anorexigenic effects of CRH are likely mediated directly through binding to low-affinity CRH type 2 (CRH2) receptors in the ventromedial (128) and paraventricular (129) nuclei of the hypothalamus, as neither hypophysectomy nor CRH1 receptor antagonists prevent CRH-induced anorexia in rats (130, 131). Stimulation of CRH2 receptors may be the mechanism through which leptin induces anorexic effects; leptin-stimulated release of CRH (44, 132), upregulation of CRH2 receptor mRNA in the ventromedial hypothalamus of rats (133, 134), and NPY inhibition (135, 136) all induce anorexic effects that can be prevented by CRH antagonists (137, 138).
CRH-stimulated release of ACTH from pituitary corticotrophs activates melanocortin receptors in the adrenal cortex, resulting in glucocorticoid (Cort) secretion, namely, cortisol in humans and corticosterone in rodents. Within the CNS, Cort binds to two types of receptors. The high-affinity mineralocorticoid receptor, located primarily in the hippocampus, is tonically activated and sensitive to the low Cort concentrations present under rest at the circadian trough (139). Under nonstressful conditions, mineralocorticoid receptor occupation maintains low ACTH levels, guarding Cort concentrations at a minimum to preserve metabolic homeostasis (140). The low-affinity glucocorticoid receptor, widely distributed throughout the brain, is phasically activated by higher Cort concentrations which are present during the circadian peak preceding daily activity and, particularly, during periods of stress (140–142). In response to stress, Cort binds to glucocorticoid receptors within the paraventricular nucleus, pituitary corticotrophs, and most notably the hippocampus, activating a negative feedback loop to reduce HPA-axis activity and halt further Cort secretion. This mechanism protects the brain from the detrimental effects of prolonged Cort exposure (143). Higher hippocampal glucocorticoid receptor density translates into greater sensitivity to the negative-feedback effects of circulating Cort and a faster “turning off” of the stress response (144, 145). Glucocorticoid receptors are also found in high density in adipocytes, particularly intra-abdominal visceral fat, where their activation leads to lipid accumulation (146).
The HPA-axis response to stress is intensity-dependent with the magnitude of Cort release related to the perceived severity of the stressor. At low concentrations, Cort enhances body weight gain through facilitation of visceral fat deposition (146, 147), and promotes food intake via reductions in hypothalamic CRH and increases in hypothalamic NPY (148–154). NPY-mediated increases in food intake and weight gain depend upon circulating Cort; NPY infusions fail to increase food intake and body weight in adrenalectomized rats unless these animals are co-treated with synthetic Cort (155). These orexigenic effects are counteracted by a Cort-induced increase in Ob gene expression and plasma leptin levels (156–158). Leptin also acts at the adrenal level to dampen further Cort release (159, 160). In the absence of Cort, the anorexigenic effect of leptin is maximized: leptin-induced decreases in body weight are greater and longer lasting in adrenalectomized animals and are dose-dependently reduced by exogenously administered Cort (161). Adrenalectomy also alleviates obesity in leptin-deficient ob/ob and db/db mice (162, 163).
In contrast to mineralocorticoid receptor occupancy at low concentrations, high Cort concentrations increase glucocorticoid receptor occupancy to produce dramatically different effects on feeding behavior. High-dose Cort decreases body weight (164–166), feeding efficiency (i.e., body weight gain per kcal of food intake; 147), and food intake (165), likely through increases in leptin secretion and upregulation of ventromedial hypothalamic CRH2 receptors (165). The weight reduction associated with glucocorticoid receptor stimulation is more pronounced in lean, than in fat, body mass. This results in an increased proportion of fat to lean tissue, particularly around the abdomen (147, 164, 167), and may account for the higher proportion of visceral to subcutaneous fat mass in anorexics (168). In sum, the modulation of feeding peptide systems by acute or chronic stress provides a mechanism whereby stress may increase the propensity to develop an eating disorder.
4.2 Stress Manipulations
In animal models, mild stressors such as brief footshock (169), mild tail pinch (170), bursts of noise (171), temporary restraint or handling (172), short-term social stress (173), saline injection (174), and a small amount of wheel running (175) all increase food intake and/or body weight. These acute stressors produce transient perturbations in the glucocorticoid circadian rhythm and only slight increases in daily mean Cort levels (140). Chronic stressors of greater intensity have the opposite effect: prolonged footshock (169, 176), repeated tail pinch (170), sustained loud noise (177), extended immobilization (178, 179), 23 h/day social separation (180), long-term social stress (173), and shifting animals from individual to paired housing (181) all decrease food intake and/or body weight. These moderate stressors produce significant elevations in Cort so that mean daily levels are increased fivefold, and glucocorticoid receptor occupation is chronic (140). Given the differential effect on food intake and body weight, acute stressors are commonly used to model bulimia and binge eating, whereas chronic stressors are incorporated into animal models of anorexia.
4.3 Activity-Based Anorexia Model
The activity-stress (182) or activity-based anorexia paradigm (183, 184) is the best established animal model of anorexia. This protocol involves unlimited running-wheel access (∼22–23 h daily), combined with limited food access (∼1–2 h daily). In both humans and rodents, running increases CRH, ACTH, and Cort secretion (185–193), particularly when it is combined with food restriction (194, 195). Early life events that heighten HPA-axis reactivity to stress increase susceptibility to activity-based anorexia (196, 197) and are thought to precipitate the disorder in humans (198). In the activity-based anorexia model, animals exhibit suppressed food intake during the once-daily meal, lower than normal body weight, and hyperactivity. These symptoms become more severe across days (183, 199) and, in the absence of intervention, may lead to death (200).
The activity-based anorexia model replicates many of the core behavioral and physiological characteristics of anorexia. For example, weight loss, food intake suppression, and hyperactivity are more pronounced in young (201) and in female (197, 202) animals subjected to this protocol. Anorexics exhibit increased restlessness and excessive exercise that correlates with decreases in leptin levels (203). In females, this leads to hypothalamic-gonadal dysfunction and amenorrhea (204). In the activity-based anorexia model, estrous cyclicity is lost as activity levels increase (205) and leptin administration suppresses starvation-induced increases in wheel running (206). Wheel-running animals show increased hypothalamic 5-HT (207) which, through stimulation of hypothalamic CRH release (208), has been proposed to mediate stress-induced anorexia (130). The role of 5-HT in anorexia nervosa is well documented (8), providing further support for the validity of the activity-based anorexia model.
Finally, the activity-based anorexia model appears to mimic changes in cognitive function that develop with this disorder. Upon initial loss of 10–15% body weight, anorexia nervosa patients are cheerful, energetic, and mentally alert; with further weight loss, fatigue, irritability, and cognitive dysfunction ensue (209–212). In animals, wheel running (207) and moderate food restriction (213) improve cognitive performance, as measured in a spatial-learning task, whereas extreme food deprivation leads to deficits in performance (213). This suggests that the activity-based anorexia model may replicate some of the cognitive deficits displayed by anorexic patients. Assessment of other cognitive dysfunctions prevalent in anorexia nervosa, such as attentional set-shifting deficits and obsessive-compulsive traits, would add to the validity of this model. Given that these tests of animal cognition are already well-established (214, 215), this area of research could progress quickly.
4.4 Binge Eating Models
Animal models of binge eating incorporate the phenomenon, well documented in humans, that stress-induced increases in feeding are magnified in the presence of calorie-laden snack foods (216). Consumption of high-fat, high-sugar “comfort” foods may occur because stress-induced opioid release suppresses HPA-axis activity (217). Intake of comfort foods sustains opioid release and increasingly inhibits HPA-axis activity (218, 219), creating a circle of events whereby humans and other animals may use comfort foods to “self-medicate” against stress (123). High dietary restraint (216), which in women is correlated with hypercortisolemia (220–223), and Cort hyperreactivity (224, 225) render individuals particularly susceptible to stress-induced bingeing. Eating comfort foods reduces Cort response to stress (226) and provides short-term alleviation of negative emotions (227). In rats, voluntary and periodic intake of food with high fat and high sugar content blunts HPA-axis reactivity by lowering hypothalamic CRH mRNA expression and reducing ACTH and Cort responses to stress (228–232). In contrast, animals that only have access to a high-fat diet exhibit increases, rather than decreases, in HPA-axis activity (233–235). The high-fat-only diet appears to act as a nutritional stressor, highlighting the importance of intermittent and voluntary intake of high-fat food in stress reduction.
Corwin and colleagues developed an animal model of binge eating disorder based on clinical findings that human binge eating occurs in the absence of hunger (236) and is directed toward high-fat foods that are deemed “forbidden” and therefore self-restricted (237). In addition to unlimited chow, rats are given limited access (e.g., 2 h) to high-fat vegetable shortening, multiple times per week for several weeks. Bingeing develops over time in that rats increase their intake of fat when it is available and restrict their intake of chow when fat is unavailable (238, 239). This behavior mimics the binge/compensation pattern typical of binge eating disorder and bulimia. Although bingeing on highly palatable food may alleviate anxiety and reduce HPA-axis activity in the short term, anxiety behaviors are increased in bingeing animals over the long term (240, 241). This also characterizes bulimic and binge eating patients who experience increased anxiety following binges once the disorder is established.
A separate model of binge eating uses a restriction-refeeding/stress protocol to model dietary restriction and stress as precipitating factors in this disorder (242, 243). Rats that experience repeated food restriction and refeeding, analogous to human “yo-yo dieting,” binge on high-fat, high-sugar food in the absence of hunger, long after restriction has ended (244). When food restriction-refeeding cycles are followed by a mild footshock, bingeing on highly palatable food is initiated sooner and is more dramatic (245). Bingeing is also more profound when cycles are introduced during the “adolescent” period in rats that experienced low levels of early-life maternal care (246), known to heighten HPA-axis reactivity to stress (247). Repeated fasting, stress, and bingeing on foods rich in sugar and/or fat decrease POMC and CRH2 receptor expression in the arcuate and ventromedial hypothalamus, respectively, increase NPY and Cort activity, and alter functioning of 5-HT, dopamine, and opioid systems in rats (165, 248–256). Disruptions in these systems are characteristic of both binge eating disorder and bulimia (8, 89, 257), providing further face validity to the restriction-refeeding/stress model.
4.5 Obesity Models
As noted previously, most animal models of obesity involve genetic variations that alter food intake and/or body weight. Chronic, mild stress can also produce obesity in animals and, like humans, this effect is associated with HPA dysregulation culminating in heightened CRH activity in the paraventricular nucleus, ineffective glucocorticoid feedback, and sustained HPA-axis stimulation (141, 165, 255, 258–262). Obese humans show elevated Cort secretion and altered Cort clearance that result in prolonged adipocyte glucocorticoid receptor activation, excess deposition of intra-abdominal fat, and leptin resistance which, together, increase obesity (146, 161, 263–266). Women with increased central fat distribution, as measured by waist-to-hip ratio, exhibit HPA-axis hyperactivity, secrete more cortisol during a laboratory stressor, and report more chronic stress compared to women with low waist-to-hip ratios (267–270). In genetically obese mice and rats, the HPA axis is hyperactive; expression of CRH2 receptor mRNA is reduced in the ventromedial hypothalamus (271, 272) whereas CRH1 receptor and hypophysiotropic CRH neuron activity is increased (134). Cort levels are elevated (273) and correspond with increased NPY mRNA content in the arcuate and paraventricular nuclei (274). These animal models therefore add credence to the idea that, although genetic makeup influences susceptibility to obesity, environmental (i.e., stress) factors play a large role in regulating expression of these genes.
Finally, some animal models of obesity incorporate developmental processes by examining how early life experiences may render animals prone to later-life obesity (275). During gestation and early preweaning, maternal consumption of a high-fat diet increases the body fat of pups at weaning (276, 277), particularly in rat lines selectively bred for diet-induced obesity (278). This is paralleled in humans as obesity during pregnancy more than doubles the likelihood of childhood obesity (279). Perhaps counterintuitively, maternal malnutrition, specifically during development of hypothalamic feeding centers in early to mid-pregnancy, increases the risk of adult obesity (280). In rats, gestational malnutrition may increase metabolic efficiency as developing animals adapt to an insufficient nutrient supply (281); with food abundance in adulthood, these adaptations may lead to increased body weight gain (282–285). Postnatally, rat pups raised in small litters exhibit adult obesity due to increased milk consumption preweaning and/or increased metabolic efficiency (286–289). Increased energy intake in human infants is associated with increased body weight in childhood and the propensity for obesity (290–292). These studies highlight the fact that environmental factors have a significant impact on body weight and adiposity and must be considered in conjunction with genetic predispositions and environmental stressors in an animal model of obesity.
5 Conclusions
Our discussion of animal models of eating disorders focused on models based on genetic alteration and those based on stressful events. The first analysis pointed to important roles for leptin and the melanocortin system in obesity, AgRP, 5-HT, estrogen, and CB1 receptor signaling in anorexia, and MC4 receptor signaling in binge eating. In general, however, most animal models based on genetic alterations have limited applicability to humans, at least to date. Those based on stressful events appear more promising in that they more accurately reproduce alterations in feeding and neuroendocrine function that are characteristic of each disorder. The next obvious step is to combine the two approaches to determine how genetic alterations and stressful events interact to produce maladaptive eating and physiological changes. Both processes clearly impact eating disorders in humans, a fact which should be reflected in animal models.
In addition to feeding and physiological changes, cognitive dysfunction is a primary characteristic of most eating disorders. This includes exaggerated food obsessions, distortions in body image, compulsive food restriction, and/or the inability to control food intake. Indeed, approximately 15% of anorexic patients and 21% of bulimic patients meet criteria for obsessive compulsive disorder (293). The restricting subtype of anorexia nervosa is typically associated with emotional, cognitive, and social inhibitions, including perfectionist and obsessive traits; in contrast binge eaters tend to be extroverted but emotionally labile to the point of being impulsive (294). In the absence of large, prospective studies of eating disorders, it is difficult to determine whether these cognitive changes precede or follow the development of an eating disorder. It is also not clear how cognitive factors would be manifested in an animal model of obesity as these have not been studied in humans. Nonetheless, the fact that disrupted feeding patterns and cognitive changes co-occur in most eating disorders suggests that animal models should include assessments of both functions.
Eating disorders are also associated with altered affective states including heightened anxiety, restlessness, and agitation in anorexia nervosa (209, 211), and a high comorbidity with mood disorders in bulimia nervosa (295). Again, the face validity of an animal model would be strengthened substantially if it reproduced one or more of these affective changes. Many of these affective and cognitive changes also typify drug addicts, adding further credence to the proposed connection between eating disorders and drug addiction (3). Indeed, evidence is accumulating that alterations in neural systems controlling reward, inhibition, motivation, and emotion contribute to maladaptive behaviors in both drug addiction and obesity (296). Whether these can be extended to other eating disorders remains an open question, one that animal research is primed to answer.
References
1.
Quadflieg N, Fichter MM (2003) The course and outcome of bulimia nervosa. Eur Child Adolesc Psychiatry 12:99–109
2.
Steinhausen H (2002) The outcome of anorexia nervosa in the 20th century. Am J Psychiatry 159:1284–1293PubMed
3.
Volkow ND, Wise RA (2005) How can drug addiction help us understand obesity? Nat Neurosci 8:555–560PubMed
4.
Wise RA (1982) Common neural basis of brain stimulation reward, drug reward, and food reward. In: Hoebel BG, Novin D (eds) The neural basis of feeding and reward. Haer Institute, Brunswick, ME, pp 445–454
5.
Garfinkel PE (1974) Perception of hunger and satiety in anorexia nervosa. Psychol Med 4:309–315PubMed
6.
Ogden CL, Carroll MD, Curtin LR, McDowell MA, Tabak CJ, Flegal KM (2006) Prevalence of overweight and obesity in the United States, 1999–2004. J Am Med Assoc 295:1549–1555
7.
American Psychiatric Association. Diagnostic and statistical manual of mental disorders. Washington, DC: 1994.
9.
Peruzzo B, Pastor FE, Blázquez JL et al (2000) A second look at the barriers of the medial basal hypothalamus. Exp Brain Res 132:10–26PubMed
10.
Cone RD (2005) Anatomy and regulation of the central melanocortin system. Nat Neurosci 8:571–578PubMed
11.
Ellacott KL, Cone RD (2006) The role of the central melanocortin system in the regulation of food intake and energy homeostasis: Lessons from mouse models. Philos Trans R Soc Lond B Biol Sci 361:1265–1274PubMed
12.
Kishi T, Aschkenasi CJ, Lee CE, Mountjoy KG, Saper CB, Elmquist JK (2003) Expression of melanocortin 4 receptor mRNA in the central nervous system of the rat. J Comp Neurol 457:213–235PubMed
13.
Chen H, Charlat O, Tartaglia LA et al (1996) Evidence that the diabetes gene encodes the leptin receptor: Identification of a mutation in the leptin receptor gene in db/db mice. Cell 84:491–495PubMed
14.
Date Y, Kojima M, Hosoda H et al (2000) Ghrelin, a novel growth hormone-releasing acylated peptide, is synthesized in a distinct endocrine cell type in the gastrointestinal tracts of rats and humans. Endocrinology 141:4255–4261PubMed
15.
Kojima M, Hosoda H, Date Y, Nakazato M, Matsuo H, Kangawa K (1999) Ghrelin is a growth-hormone-releasing acylated peptide from stomach. Nature 402:656–660PubMed
16.
Kamegai J, Tamura H, Shimizu T, Ishii S, Sugihara H, Wakabayashi I (2001) Chronic central infusion of ghrelin increases hypothalamic neuropeptide Y and agouti-related protein mRNA levels and body weight in rats. Diabetes 50:2438–2443PubMed
17.
Wren AM, Small CJ, Abbott CR et al (2001) Ghrelin causes hyperphagia and obesity in rats. Diabetes 50:2540–2547PubMed
18.
Shintani M, Ogawa Y, Ebihara K et al (2001) Ghrelin, an endogenous growth hormone secretagogue, is a novel orexigenic peptide that antagonizes leptin action through the activation of hypothalamic neuropeptide Y/Y1 receptor pathway. Diabetes 50:227–232PubMed
19.
Bulik CM, Sullivan PF, Wade TD, Kendler KS (2000) Twin studies of eating disorders: A review. Int J Eat Disord 27:1–20PubMed
20.
Bulik CM, Sullivan PF, Kendler KS (2003) Genetic and environmental contributions to obesity and binge eating. Int J Eat Disord 33:293–298PubMed
21.
Rankinen T, Zuberi A, Chagnon YC et al (2006) The human obesity gene map: The 2005 update. Obesity 14:529–644PubMed
22.
Foch TT, McClearn GE (1980) Genetics, body weight and obesity. In: Stunkard AJ (ed) Obesity. W.B. Saunders, Philadelphia, pp 48–71
23.
Speakman J, Hambly C, Mitchell S, Król E (2007) Animal models of obesity. Obes Rev 8:55–61PubMed
24.
Barsh GS, Farooqi IS, O’Rahilly S (2000) Genetics of body-weight regulation. Nature 404:644–651PubMed
25.
Coleman DL (1978) Obese and diabetes: Two mutant genes causing diabetes-obesity syndromes in mice. Diabetologia 14:141–148PubMed
26.
Friedman JM, Leibel RL, Siegel DS, Walsh J, Bahary N (1991) Molecular mapping of the mouse ob mutation. Genomics 11:1054–1062PubMed
27.
Zhang Y, Proenca R, Maffei M, Barone M, Leopold L, Friedman JM (1994) Positional cloning of the mouse obese gene and its human homologue. Nature 372:425–432PubMed
28.
Garthwaite TL, Martinson DR, Tseng LF, Hagan TC, Menahan LA (1980) A longitudinal hormonal profile of the genetically obese mouse. Endocrinology 107:671–676PubMed
< div class='tao-gold-member'>
Only gold members can continue reading. Log In or Register a > to continue
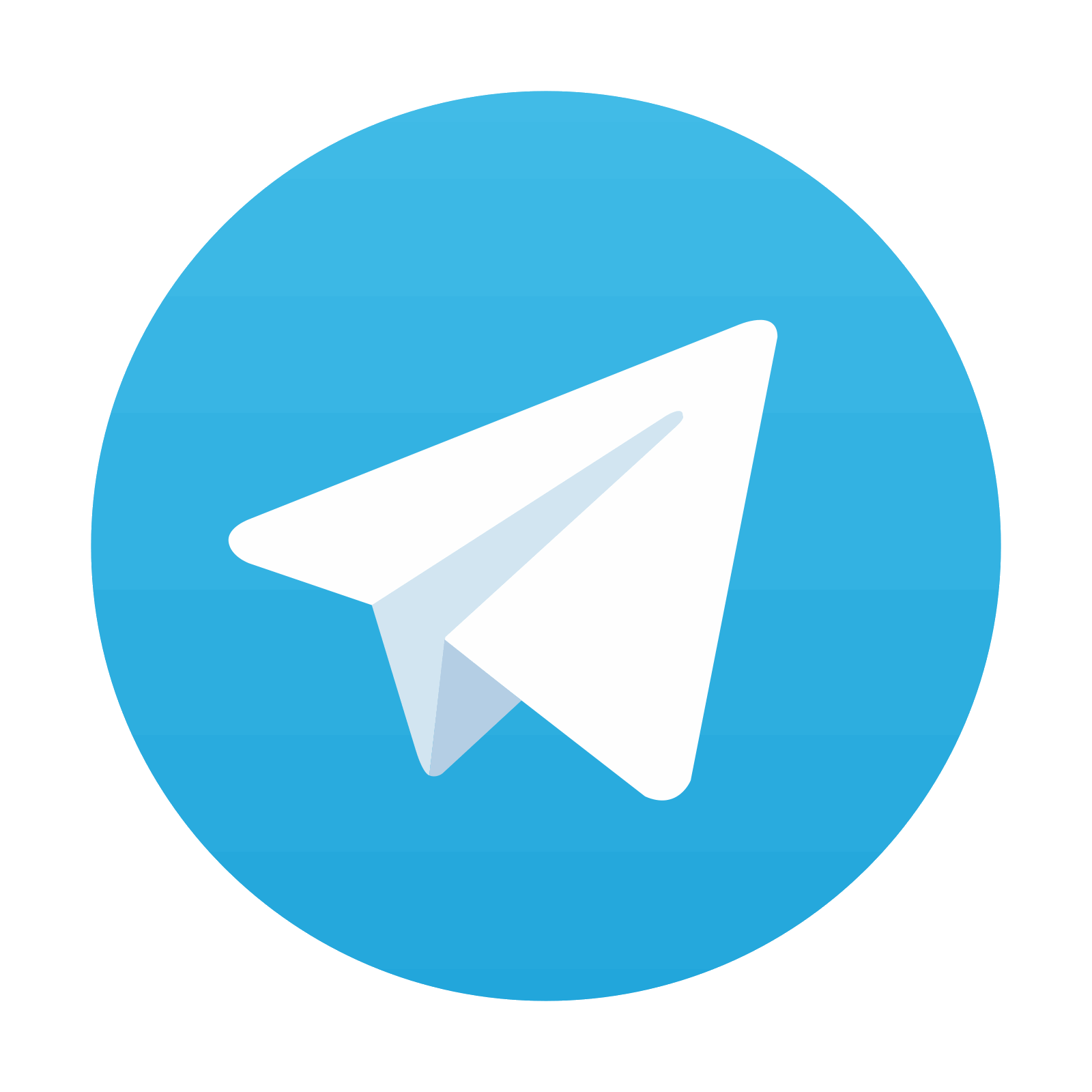
Stay updated, free articles. Join our Telegram channel
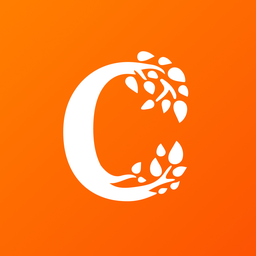
Full access? Get Clinical Tree
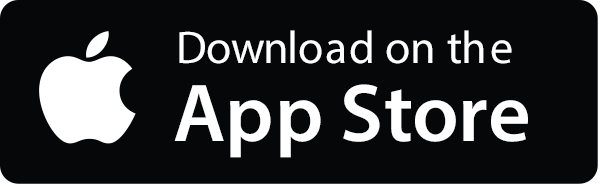
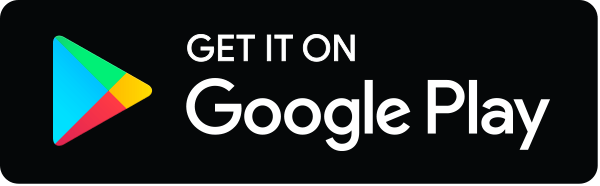