Chapter 23 Administration of an intravenous drug by constant rate infusion (CRI) allows more precise dosing to a target drug level or effect. It also eliminates the peaks and troughs of serum drug concentration that occur with bolus dosing. Many drugs used in anesthesia, including benzodiazepines, opioids, propofol, ketamine, and alpha-2 agonists, may be administered as a CRI. In addition, many vasoactive drugs used during anesthesia are administered as a CRI because of their short half-lives. For this reason, rapid calculation of CRI dosages is a necessary skill for the advanced anesthetist. CRIs may be administered via a syringe pump, or by preparation of a dilution of drug in normal saline or other appropriate isotonic fluid, which then can be administered by pump or drip set. Only diazepam is unable to be diluted in this manner, because of its adsorption to plastics and its insolubility in water. Some schemata for calculating CRI rates are provided in Box 23-1. CRIs are useful in the postanesthetic period as well, allowing the veterinarian to tailor a specific rate and dose of drug to the patient’s needs. This phenomenon is the concept behind low-flow (closed-circuit) anesthesia; at equilibrium, only enough inhalant must be supplied to make up for that lost from the system by redistribution or metabolism, and only enough oxygen must be supplied to meet the animal’s metabolic demands (approximately 5 mL oxygen/kg/min, depending on temperature and metabolic factors). Higher inhalant concentrations may have to be delivered at low flows, although the total amount of agent delivered into the circuit is significantly less than that delivered at moderate to high flows. Closed-circuit anesthesia in human beings and animals has been reviewed elsewhere.18,120,170 Vaporizers are designed and calibrated to deliver a constant concentration of anesthetic vapor over a wide variety of carrier gas flows and ambient temperatures. The carrier gas used for initial calibration of some vaporizers ranges from 21% oxygen (medical air) to 100% oxygen, depending on the manufacturer. Therefore, if N2O or medical air (primarily nitrogen) mixed with oxygen is used as the carrier gas, the output may be different than that indicated by the dial.251 Also, as carrier gas flow increases above 5 L/min, or below 0.1 L/min, the concentration of vapor delivered from the vaporizer may be significantly different from the dial setting.9 Most vaporizers are designed for the ambient temperature range typically encountered in the operating room setting. However, extreme temperatures, such as those that may be encountered in an outdoor field situation, may affect vaporizer output, usually with warmer weather increasing output and colder weather decreasing output.114 At high altitudes, because of the relatively lower atmospheric pressure, vaporizer output may be slightly higher than the dialed concentration for most vaporizers, with the exception of the desflurane vaporizer. Vaporizer Output: Output from vaporizers may be variable-bypass or measured flow. Variable-bypass is made by splitting the incoming fresh gas flow to direct a variable portion through the vaporizing chamber and the remainder through a bypass chamber. The two flows are merged before exiting the vaporizer, producing the concentration set by the dial (Figure 23-1). Variable-bypass vaporizers are the most common type of vaporizer in clinical use today. Measured-flow vaporizers use two different flowmeters, one that passes a small amount of carrier gas through the vaporizer, fully saturating this gas with anesthetic vapor, and a larger diluent flow, which does not contact any inhalant and dilutes the saturated carrier gas to the appropriate concentration for inhalation by the patient. The flows for this type of anesthetic machine are set independently, and calculations are necessary to determine the gas flows necessary to result in specific anesthetic concentrations. For these reasons, measured-flow vaporizers are rarely used in modern anesthetic practice. Methods of Vaporization: Methods of vaporization for inhalant anesthetics include flow-over, bubble-through (both of these require a vaporizer chamber), and direct injection. In flow-over vaporizers (see Figure 23-1), the carrier gas passes over a reservoir of inhalant and picks up the anesthetic vapor as it does so (the concentration of vapor being relative to the specific vapor pressure of the agent). Often, wicks are incorporated into the vaporizer to increase surface area for contact between the inhalant and the carrier gas. These are the vaporizers most commonly used in current clinical practice. Bubble-through vaporizers bubble the carrier gas through the bottom of the reservoir of anesthetic to pick up the vapor. This type of vaporizer is used in measured-flow machines such as the “copper kettle,” and is rarely used in modern anesthetic practice. Injection-type vaporizers inject an atomized spray of inhalant into the stream of the carrier gas, rapidly vaporizing the inhalant, as is done in desflurane vaporizers. Liquid anesthetic agent may also be directly injected into the anesthetic circuit by the anesthetist.30 Circle Systems: Vaporizers may be located in the anesthetic circuit or out of the circuit. In circuit vaporizers have highly variable output depending on ambient temperature, patient ventilation, and the volatility of the selected agent. They do not have temperature compensation and may incorporate wicks for the vaporization of agents with low vapor pressures (e.g., methoxyflurane). The wicks should be removed for use with more volatile agents such as isoflurane and sevoflurane.197 With an increase in respiratory minute volume, the patient will draw a greater volume of carrier gas over the vaporizer, resulting in an increase in the concentration of inhalant in the circuit. In practice, this may result in a variable anesthetic plane. In circuit vaporizers are not often used for veterinary anesthesia. Temperature Compensation: Vaporizer temperature compensation is necessary, as the vaporization of inhalant from the reservoir lowers the reservoir temperature. As high-energy molecules near the surface of the liquid break free, they take a high-energy state (heat) with them, leaving a lower net amount of energy in the liquid. As the temperature of the reservoir decreases, fewer molecules will be liberated to a gaseous state, thus lowering the output from the vaporizer. Vaporizers incorporate a variety of mechanisms to ensure that the anesthetic concentration from the vaporizer chamber remains constant over a variety of temperatures, including construction materials with a high specific heat and bimetallic valves to control the amount of bypass. Vaporizer Agent Specificity: Most out of circuit precision vaporizers are designed and calibrated for use with single agents, taking into account the vapor pressure of the specific agent. Safety mechanisms such as keyed filling devices are available on some models to ensure that they are filled with only the appropriate agent for the vaporizer. If an incorrect agent is used in an agent-specific vaporizer, this may result in a higher or lower output concentration. The vaporizer for desflurane is unique from the other vaporizers in common use today. The saturated vapor pressure for desflurane is very high, making desflurane very nearly a gas at standard temperature and pressure (STP) (the boiling point of desflurane is 74.3° F [23.5° C]), and it is stored in a pressurized bottle. A standard vaporizer would create too high of a desflurane concentration for safe use and might result in unpredictable amounts of vapor output with fluctuations in temperature. The solution has been to create an externally warmed vaporizer, which turns the desflurane from a liquid/vapor fully into a gas. The desflurane gas is then injected into the carrier gas, creating a clinically useful concentration. The vaporizer relies on external power for its function, so it must be plugged in to a power source. In addition, because desflurane vaporizes so readily, the refilling mechanism ensures a tight seal between the refilling bottle and the vaporizer to prevent any desflurane vapor from escaping.11 The rebreathing circuit is defined by the use of a CO2 absorbent to remove CO2 from the system. This absorbent is supplied in granule form (from 4 to 8 mesh in size) and typically contains a strong base in a proprietary formula that also incorporates water into the granules. For example, soda lime is a combination of sodium hydroxide, potassium hydroxide, water, and calcium hydroxide. CO2 exhaled by the patient reacts with water to form carbonic acid on the surface of the absorbent granules. The carbonic acid then dissociates to free protons and carbonate. These then associate with the strong bases to form water and calcium carbonate. Heat and water are created in the reactions. An indicator dye (ethyl violet) similar to that used with litmus paper, changes color on reaction with acid. The absorbent granules will take on a purple color as they absorb CO2 and will gradually lose their ability to absorb additional CO2. With soda lime, some regeneration of CO2 absorbent capacity may occur over time. This may allow granules that had previously changed color to lose their color change, but the regeneration is minimal, and these granules will rapidly become saturated and change color again once exposed to CO2. As a general rule, the CO2 absorbent should be changed whenever rebreathing of CO2 by the patient is seen on a capnograph. Other guidelines will vary, depending on the degree of machine usage and the average fresh gas flows used. At higher fresh gas flows, the absorbent may lose water to evaporation and become less effective for CO2 removal (this may also result in the production of other byproducts, such as carbon monoxide [CO]).97 In addition to the CO2 absorbent, the circle system (Figure 23-2) incorporates one-way valves to prevent rebreathing of expired CO2, a reservoir bag to allow positive-pressure ventilation, a pressure gauge, an adjustable pressure limiting valve (commonly called the APL, or “pop-off” valve), and breathing tubes. The common gas inlet enters the system upstream from the inspiratory valve. From there, the gas flows into the inspiratory limb of the breathing circuit, into the patient, out through the expiratory limb of the breathing circuit, through the expiratory valve, past the reservoir bag and pop-off, through the absorbent (where the CO2 is removed), and finally back through the inspiratory valve to the patient. The inspiratory and expiratory valves ensure unidirectional flow through the system. If the inspiratory valve becomes stuck open when the patient breathes out, the expired air can travel up the inspiratory limb of the circuit. On the next inspiration, the patient will rebreathe this exhaled gas, which contains CO2. If the expiratory valve becomes stuck open, the expired gas in the expiratory limb of the circuit can be inhaled on the next inspiration, causing the patient to rebreathe gas containing CO2. The rebreathing systems require relatively low fresh gas flows compared with the nonrebreathing system. The minimum oxygen flow for the rebreathing system is equal to the patient’s metabolic oxygen demand (usually estimated as 10 × body weight (kg)0.75). If the carrier gas is only oxygen and is set to equal the patient’s metabolic oxygen demand, the system is said to be functioning as a “closed” circuit, since the pop-off valve may be closed and the pressure inside the system will not change because all incoming oxygen is being metabolized. This is a highly economical system, because relatively little inhalant anesthetic will be vaporized from the vaporizer, and little oxygen will be used. Alternatively, use of a higher, standard fresh gas flow (e.g., 1 L/min), creating a semi-closed rebreathing system, allows the clinician to calculate the time constant, or the amount of time necessary to effect a change in inspired anesthetic amount with a change in vaporizer setting.229 Dead space is produced in the rebreathing system where the inspiratory and expiratory breathing tubes meet at the patient (the Y piece). In a small patient, this dead space may be sufficient to cause rebreathing of CO2. Therefore, rebreathing systems are typically reserved for patients larger than 5 kg.206 In practical terms, this is the only system that works for very large animals, as the nonrebreathing system cannot eliminate CO2 rapidly enough for very large patients. Nonrebreathing systems prevent rebreathing of CO2 by using high fresh gas flow rates. The terminology arises from the fact that rebreathing of expired gases is minimized or eliminated by high incoming fresh gas flow. As a patient breathes in, inspired gas is supplied by the common gas outlet continuously throughout the inspiratory cycle. Upon exhalation, the expired gas passes into a reservoir bag or out of the system via the adjustable pressure-limiting valve. The recommended fresh gas flow rate for an nonrebreathing system is at least three times the patient’s respiratory minute volume (MV).205 MV = Respiratory rate × Tidal volume; estimated tidal volume = 15 mL/kg.205 If the common gas outlet enters the nonrebreathing circuit close to the patient, the gas from the common gas outlet is delivered directly to the patient. The exact way that gas flows in a nonrebreathing system is determined by the organization of the system. Most nonrebreathing systems represent one of the schemata described by Mapleson.218 Because no dilution of this gas occurs, as happens in rebreathing systems, the inhalant concentration set on the vaporizer represents the inspired concentration of inhalant that is delivered to the patient. Fresh gas flows into the patient with inspiration. On exhalation, expired gases are exhausted from the system. On the next inspiration, a small amount of expired gas may enter the patient before the incoming fresh gas gets to the patient, particularly if the fresh gas flow rate is too low. Nonrebreathing circuits (Figure 23-3) offer minimal resistance to airflow and minimal dead space, making them most suitable for small patients. They also have fewer components so are technically simpler and easy to clean.205 However, nonrebreathing circuits may not be compatible with some ventilators and are wasteful because of the high fresh gas flows necessary to prevent rebreathing. Depending on the type of system, fresh gas flows from 200 to 500 mL/kg are required to prevent rebreathing. This increases the amount of inhalant vaporized and increases oxygen used by the system. Nonrebreathing circuits typically are not effective for larger patients. Patients weighing less than 5 kg are best anesthetized using a nonrebreathing system rather than a rebreathing system. Delivery of gas from the anesthetic circuit to the patient is best accomplished via an endotracheal tube. Maintenance of anesthesia with a mask may be suitable for short procedures; however, masks can leak, which is wasteful and exposes personnel to anesthetic gases. Masks do not maintain a patent airway, cannot be used to provide effective intermittent positive-pressure ventilation (IPPV), and may instill gas into the esophagus or stomach. Endotracheal intubation with an endotracheal tube allows maintenance of a patent airway, and ensures delivery of anesthetic gases to the lungs while preventing aspiration of material from the oropharynx. Anesthetic maintenance using an endotracheal tube also minimizes leakage of waste gases and allows for orofacial surgery. However, endotracheal intubation requires a sufficiently anesthetized patient for placement and may increase airway resistance, work of breathing, and dead space. Endotracheal intubation requires technical expertise and can result in damage to the larynx, trachea, and/or lungs.5,45,147,234 Endotracheal tubes may become kinked or obstructed, causing airway obstruction. Laryngeal mask airways may be used in animals and are designed to fit over the larynx.49,335 A seal is maintained by inflating a cuff around the laryngeal mask airway. The laryngeal mask airway is less traumatic than an endotracheal tube but may be more easily displaced, and provision of IPPV may be difficult.335 Most endotracheal tubes have a Murphy eye near the tip, which allows for continued airflow should the tip of the tube become obstructed (Figure 23-4). Endotracheal tubes are commonly composed of rubber, silicone, or polyvinyl chloride (PVC). Rubber tubes are opaque and stiff, silicone tubes are soft but may require the use of a stylet because of their lack of stiffness, and PVC tubes are clear and stiff. Guarded endotracheal tubes have an embedded metal spiral, which helps prevent kinking, and are particularly useful in orofacial surgery, but they are incompatible for use with magnetic resonance imaging. Figure 23-4 A Murphy-type endotracheal tube, highlighting the presence of the Murphy eye at the distal portion. Failure to establish and maintain a patent airway may lead to hypoxemia. In a patient breathing room air, hypoxemia will develop within 30 seconds of apnea or airway obstruction, whereas a patient previously breathing 100% oxygen may not become hypoxemic for upward of 5 minutes after onset of apnea.187 Patients with facial trauma, myositis, laryngeal masses, and similar conditions may be challenging to intubate orotracheally. Because induction of anesthesia is frequently accompanied by apnea or hypoventilation, such patients should be preoxygenated by placing the muzzle or head in a mask and allowing them to breathe100% oxygen for 5 minutes. An oxygen flow rate into the mask of 4 to 5 L/min is adequate for preoxygenation of most small-animal patients. This saturates the alveoli with oxygen, providing a reservoir of oxygen in the alveoli, and particularly within the functional residual capacity, in the event of apnea, upper airway obstruction, or delayed intubation. Use of a stylet within the endotracheal tube may facilitate placement. Careful passage of a long catheter (e.g., a flexible red-rubber catheter) into the trachea to serve as a guide may be necessary. The catheter may be placed through the Murphy eye to allow the endotracheal tube to be subsequently threaded into the trachea. The anesthetist may also use this catheter to insufflate oxygen during the intubation process. Commercially available airway exchange catheters are available for this use or for simplifying reintubation.342 Difficult Intubation: Difficult tracheal intubation may occur for a variety of reasons. Patients with myositis may be unable to open their mouths to allow direct laryngoscopy or intubation. In these patients, progressively opening the mouth by stacking tongue depressors horizontally between the top and bottom incisors can increase the size of the oral opening, allowing direct laryngoscopy. The use of small stylets to facilitate intubation as described above is another method to achieve a secure airway in patients unable to open their mouths. If nasopharyngeal swelling or a mass prevents direct visualization, fiberoptic or semi-rigid endoscopes may be used to identify and intubate the trachea, providing a pathway for endotracheal tube placement. A through-the-needle jugular catheter may be introduced through a proximal tracheal ring and passed orad into the oral cavity, where the endotracheal tube can be passed over it and into the trachea. If the airway cannot be secured via orotracheal methods, a tracheostomy may be performed to secure and maintain the airway. Oxygen is the most common carrier gas for inhalant anesthetics. Use of oxygen as the carrier gas ensures maximal oxygen delivery to the patient and is the standard for veterinary anesthesia machines. Use of 100% oxygen may result in relatively more alveolar collapse than 40% oxygen because the nitrogen contained in air/oxygen mixtures is not readily absorbed from the alveoli. The nitrogen thus provides structural support (the nitrogen “scaffold”) to the alveolus. Because oxygen is rapidly absorbed from the alveoli, an alveolus filled with 100% oxygen will gradually collapse as that oxygen is removed by the pulmonary blood flow (absorption atelectasis).305 Oxygen is supplied to the anesthesia machine via portable tanks or piping from a central source. Larger tanks, liquid oxygen, and oxygen concentrators are more cost-effective than smaller tanks. These tanks are all pressurized, and great care should be taken when handling them. Connections should not be forced when pressurized gases are handled, and tanks should be transported attached to a stable surface, and secured whenever upright. The volume of oxygen in a tank is directly related to the pressure and size of the tank, so that a tank with half the starting pressure will also have half the amount of oxygen remaining in the tank. This is not the case for all medical gases; supply information for commonly used medical gases is shown in Table 23-1. Table • 23-1 Anesthetic Gases and Partial Pressures of Each Gas in Commonly Available Cylinders78* *The cylinders should always be color-coded and labeled. A pin index system, different for each gas, is also standard to prevent misuse of the gases. From Dorsch JA, Dorsch SE: Understanding anesthesia equipment, ed 4, Philadelphia, 1995, Lippincott Williams & Wilkins. Oxygen increases the flammability and volatility of existing chemicals. For example, ether in room air causes cool flames, but in 100% oxygen causes explosions. Accumulation of high concentrations of oxygen due to inappropriate connections can result in catastrophe in the face of a spark or use of some surgical lasers or electrocautery. High oxygen concentrations are contraindicated when some surgical lasers are used for surgery adjacent to the endotracheal tube, and shielding tape is available to protect against inadvertent laceration of the tube and oxygen leakage, which would result in fire.103 Helium may be combined in a 70 : 30 ratio with oxygen to protect against fires caused by laser ignition of anesthetic carrier gas, although this requires a specially calibrated additional flowmeter.259 Errors in the connection of gases are minimized by use of the pin index safety system, the diameter index safety system, and color coding of the tanks of gas. The pin index safety system (Figure 23-5) uses protrusions on the machine, which must line up with depressions on the tank or connector. The diameter index safety system uses different diameter adaptors on hoses for each type of gas. Incorrect or forced connections can result in elevated levels of N2O in the circuit (a hypoxic gas mixture), leaks, unknown oxygen concentration and delivery, and the unknown presence of other gases. Pressure Regulation: An internal pressure regulator on the anesthesia machine reduces the carrier gas pressure from that in the tank or wall outlet to 50 pounds per square inch (PSI). The flowmeter further reduces the oxygen flow to clinically appropriate levels. Most oxygen flowmeters on small-animal anesthetic machines deliver up to 4 L/min. The flow rate is read from the top of a bobbin or the middle of a ball that floats alongside the flowmeter scale. From the flowmeter, oxygen travels to the vaporizer. The oxygen flush valve delivers oxygen, which bypasses the flowmeter and vaporizer and is consequently delivered at high pressure. The oxygen flush valve should not be used with nonrebreathing systems because of the risk of significant barotrauma from the high flow rate (30 to 50 liters per minute [LPM]). Gas Scavenging: Inhalant anesthetics are potential toxins and pollutants; therefore personnel and environmental exposure should be minimized.61,196 No causal relationship between exposure to the newer volatile inhalant anesthetics and occurrence of disease among health care providers has been proven, yet caution should be exercised in the handling of waste gases.181 Some evidence suggests that chronic exposure to N2O may result in decreased reproductive health among operating room personnel.160 Oxygen delivery to tissues is dependent on both blood flow through the body and the oxygen content of the arterial blood being carried. Blood flow is generated by cardiac output, the amount of blood pumped by the heart per minute (a product of heart rate and stroke volume). In the awake patient, oxygen delivery to tissues is maintained by alterations in cardiac output, in addition to local tissue factors. In the anesthetized patient, sympathetic responses are blunted, necessitating monitoring and possibly intervention by the anesthetist. The oxygen content of arterial blood is largely dictated by the hemoglobin concentration. Decreases in hemoglobin lead to a linear decrease in the oxygen content of arterial blood. Profound hypoxemia can also decrease the oxygen content of arterial blood, but this effect is far less dramatic than the effect of anemia (Figure 23-6). In the face of suboptimal oxygen delivery to tissues, anaerobic metabolism occurs, which produces dramatically less adenosine triphosphate (ATP) for a given amount of glucose than aerobic metabolism, and results in the production of lactate and acid (H+) as byproducts. Prolonged tissue hypoxia results in an insufficient supply of ATP to power important enzymes such as the sodium-potassium ATPase, which produces energy to maintain the cell electrochemical gradient. Without the electrochemical gradient, cell swelling occurs and can lead to cell death. Cellular dysfunction due to prolonged hypoxia may lead to organ failure. In practical terms, the brain, kidney, and heart are most susceptible to tissue hypoxia, and decreased oxygen delivery to tissues most commonly manifests as dysfunction in any or all of these organs. Decreased blood flow to the liver may alter the metabolism of anesthetic drugs and result in prolonged clearance of some drugs. In addition to monitoring the above body systems, the patient must be rendered unconscious, be free from pain, and have muscle relaxation for general anesthesia to commence successfully. A poorly anesthetized patient may have sympathetic stimulation or movement in response to a procedure, which may impair the ability of the surgeon to complete the procedure. Excessive sympathetic stimulation may lead to cardiac arrhythmias. Pain causes myriad adverse consequences, and analgesia must be provided during any procedure that induces pain (Table 23-2). Anesthetic depth should be monitored regularly on the basis of clinical signs. A medium-light to medium depth is ideal for most procedures, allowing the surgeon to perform the task without inducing an excessive degree of homeostatic depression. Perioperative analgesic regimens and strategies are discussed further in this chapter and also in Chapter 22. Normal values for systolic arterial pressure, mean arterial pressure, and diastolic arterial pressure vary with species. In awake dogs and cats, the normal values are typically written as SAP/DAP (MAP) in mm Hg and are 125/85 (105).311 Values in anesthetized animals are typically lower. When the mean arterial pressure is less than 60 mm Hg, perfusion and oxygen delivery to the kidney and brain are likely insufficient to meet the requirements for aerobic metabolism.86 Prolonged hypotension can result in cerebral and renal ischemia, which manifests as alterations in brain function (often blindness, prolonged recovery, and mental dullness) or acute renal failure. Because the heart fills in diastole, a diastolic arterial pressure less than 40 mm Hg indicates poor coronary artery perfusion, and possible cardiac ischemia.99 Indirect Blood Pressure Measurement: The Doppler method of indirect blood pressure measurement relies on the recognition of a change in sound produced as blood passes under the Doppler crystal. The crystal emits a sound wave and, as that wave is reflected by the blood, receives the change in signal created by the velocity of the flowing blood. Although a Doppler crystal may be placed over any artery for assessment of blood flow, to measure blood pressure, it must be placed over a peripheral artery. The most commonly used arteries are the radial artery in the forelimb, the plantar metatarsal artery in the hindlimb, and the coccygeal artery in the tail. The Doppler crystal is placed over the artery and is secured with tape. Proximal to the crystal, an occlusive inflatable cuff is secured around the limb or tail. The width of the cuff should be equal to 40% to 60% of the circumference of the limb or tail. A cuff that exceeds this recommendation will result in an artificially low value, and the use of a cuff that is too small will result in an artificially high value. A cuff that is loose and is not snugly secured on the patient will also result in an artificially high value. The inflatable bladder of the cuff should be placed over the artery to be occluded. The cuff is then connected to a sphygmomanometer and is inflated until no sound can be heard from the Doppler. Air is slowly released from the cuff until sound can be heard again—this is the systolic arterial pressure. A change in the tone of the signal may be appreciated as the cuff continues to be deflated and may be interpreted as the diastolic arterial pressure. The diastolic arterial pressure can be challenging to define with the Doppler method, and therefore the systolic arterial pressure is the only reliable value obtained with the Doppler.311 In cats, the Doppler tends to underestimate the systolic arterial pressure by up to 25 mm Hg.51 In dogs, the Doppler reading correlates well with the direct arterial pressure reading in most anesthetized patients.53 The Doppler sound pulse is not affected by tachyarrhythmias, bradyarrhythmias, or irregular heartbeats. A variation on the Doppler method uses the waveform produced by a pulse oximeter device to interpret the point of occlusion of the artery. This is known as photoplethysmography. The cuff is inflated by the sphygmomanometer until the waveform of the pulse oximeter disappears. Air is then released from the cuff until a waveform appears—this is the systolic arterial pressure. This technique relies on a good pulse oximeter waveform with placement of the pulse oximeter probe distal to the cuff. Typically, only the webbing of the front and hind feet is suitable for this purpose. Photoplethysmography seems to offer no advantage over traditional Doppler methods for indirect blood pressure measurement.51 The oscillometric method of indirect pressure measurement is an automated system. A cuff is attached in a similar fashion to the Doppler method (using the same sizing guidelines). The machine then inflates the cuff to a sufficient pressure to occlude the artery. The cuff is then either slowly deflated or deflated in steps by the machine. As oscillations in the cuff occur as a result of the return of pulsatile blood flow through an artery partially collapsed by the cuff pressure, those oscillations are sensed by the machine and interpreted as the systolic arterial pressure. Oscillations with the greatest amplitude are interpreted as the mean arterial pressure, and the point of absence of oscillations is interpreted as the diastolic arterial pressure. Some machines only measure the mean arterial pressure and systolic arterial pressure and calculate the diastolic arterial pressure, and all have proprietary algorithms for determining pressure. In cats, oscillometric devices tend to underestimate systolic arterial pressure but are relatively precise for mean and diastolic arterial pressure.51 In dogs, oscillometric devices tend to underestimate systolic, diastolic, and mean arterial pressures, although this bias is changed in the face of hypotension and other disease states.311 In both species, the trend of readings is consistently correlated with the direct arterial pressure. Because the machine requires regular oscillations to determine pressure, oscillometric machines may fail to generate a reading in the presence of irregular heart rhythms, or tachyarrhythmias or bradyarrhythmias.
Anesthesia Principles and Monitoring
Anesthesia Principles and Monitoring
Anesthetic Drug Delivery
Vaporizers
Anesthetic Circuits
Rebreathing System
Closed and Semi-Closed Circuit Rebreathing System
Nonrebreathing Systems
Endotracheal Tubes
Intubation and Preoxygenation
Fresh Gas
Anesthetic Monitoring Equipment
Anesthetic Depth
Blood Pressure Monitoring
Stay updated, free articles. Join our Telegram channel
Full access? Get Clinical Tree
Anesthesia Principles and Monitoring
Only gold members can continue reading. Log In or Register a > to continue
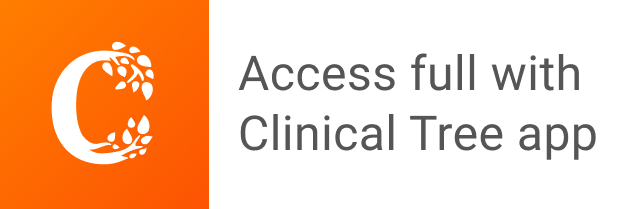