Chapter 19 Adrenocortical Function II. PHYSIOLOGY OF ADRENOCORTICAL HORMONES E. Metabolic Breakdown and Excretion F. Steroid Receptors and Actions G. Physiological Variation, Stress, and the Immune System IV. ASSESSMENT OF ADRENOCORTICAL FUNCTION A. Routine Laboratory Diagnostics B. Tests of Basal Adrenocortical Function D. Tests for Differential Diagnosis E. Collection and Handling of Samples In mammals, the adrenal glands are bilateral structures located craniomedial to the kidneys. The adrenal is, in fact, made up of two glands of different embryological origin. The center of the gland, the medulla, comprises coalesced chromaffin cells of neuroectodermal origin that secrete epinephrine or norepinephrine. The surrounding adrenal cortex arises from mesoderm and can be divided histologically into three zones: (1) zona glomerulosa (or arcuata), (2) zona fasciculata, and (3) zona reticularis. The cells of the zona glomerulosa are arranged in ovoid groups immediately inside the connective tissue capsule. The region may be particularly obvious (e.g., sheep) or difficult to discern (e.g., small rodents). The zona fasciculata accounts for most of the adrenal cortex (>60%) and appears histologically in radial lines. The inner zona reticularis comprises networks of cell cords surrounding the large blood sinuses. A notable feature of the ferret adrenal gland is that islands of cortical cells can be found among cells of the medulla. These cells resemble those of the inner part of the zona fasciculata (Holmes, 1961). In birds, the adrenal glands are also separate, encapsulated organs. The glands lie cranial to the kidney, often wholly or partly covered by the gonads. The adrenocortical and chromaffin tissues are intermingled to varying degrees, depending on the species. The basic unit of avian adrenocortical tissue is a cord of a double row of parenchymal cells, with their long axes in the transverse plane of the strand. The cords radiate from the center of the gland, branch, and anastomose frequently. Groups of chromaffin cells lie between the cords and are also ensheathed within the connective tissue reticulum (Chester-Jones, 1987). Blood flow of the adrenals is centripetal. In species with separated medulla and cortex, this results in high medullary corticoid concentrations that induce an enzyme in the chromaffin cells, converting norepinephrine to epinephrine. Therefore, species with anatomically separated medulla and cortex predominantly secrete epinephrine and species with intermingled chromaffin and adrenocortical tissue secrete predominantly norepinephrine (Marks, 1983). The secretion of the mammalian adrenal cortex comprises three main categories of hormones, which can be related to some extent to the above-described anatomical zonation. The zona glomerulosa produces mineralocorticoids (aldosterone and deoxycorticosterone), which maintain salt balance. The cells of the zona fasciculata secrete glucocorticoids (cortisol and corticosterone), which are primarily involved in carbohydrate metabolism. The third category of adrenocortical hormones, the androgens (e.g., androstenedione), is produced in the zona reticularis. This zone to a minor degree also secretes glucocorticoids and other hormones such as progesterone and estrogens. FIGURE 19-1 Numbers of the carbon atoms and letters designating the rings of the pregnenolone molecule (IUPAC-IUB, 1989). In birds aldosterone and corticosterone are the main corticosteroids secreted. Zonation of the avian adrenal is less clear than in the mammalian adrenal. However, the outer subcapsular cells, looping in a manner similar to the zona glomerulosa, appear to be the predominant aldosterone secretors. The cells reaching toward the central part of the gland form corticosterone (Kime, 1987). The adrenal steroids contain as their basic structure a cyclopentanoperhydrophenanthrene nucleus consisting of three six-carbon rings (A, B, and C) and a single five-carbon ring (D). The letter designations for the carbon rings, and the numbers of the carbon atoms are shown for pregnenolone (Fig. 19-1), a key biosynthetic intermediate. The Greek letter Δ indicates a double bond, as does the suffix -ene. The position of a substituent below or above the plane of the steroid ring is indicated by α and β, respectively. The α substituent is drawn with a broken line (e.g.–OH) and the β substituent with a solid line (e.g.-OH). The C18 steroids, which are devoid of a side chain at C-17 and have a substituent at C-18, are estrogens. The C19 steroids, which have substituent methyl groups at positions C-18 and C-19, are androgens (see also Fig. 19-1) (IUPAC-IUB, 1989). Steroids that have a ketone group at C-17 are termed 17-ketosteroids. The C21 steroids, the corticosteroids and progestagens, are those that have a two-carbon side chain (C-20 and C-21) attached at C-17 and in addition have substituent methyl groups at C-18 and C-19. The C21 steroids that also possess a hydroxyl group at position 17 are termed 17-hydroxy-corticosteroids and may have predominantly glucocorticoid properties. Cholesterol, derived from food and from endogenous synthesis via acetate (Fig. 19-2), is the principal starting compound in steroidogenesis. The adrenal gland is enriched in receptors that internalize low- and high-density lipoproteins. This uptake mechanism increases when the adrenal is stimulated and provides the major cholesterol source. Subsequent steps occur in the mitochondrion or at the endoplasmic reticulum. Two classes of enzymes are involved in the synthesis of steroids, the cytochrome P450 (CYP) heme-containing proteins that catalyze mainly hydroxylation reactions and the hydroxysteroid dehydrogenases (HSD) that are involved in oxidation and reduction reactions (Payne and Hales, 2004). The human CYP enzymes are written with capitals, whereas in mice lowercase is used. For other species no rules are given, but we will use here the abbreviations as used for human. The precursor cholesterol has a side chain that is cleaved by CYP11A1 resulting in formation of pregnenolone (Fig. 19-2). Mice defective in the Cyp11a1 gene produce no steroids, survive during embryogenesis, but die after birth (Hsu et al., 2006). The zonal difference in adrenocortical hormone production is due to two steroidogenic enzymes. The mitochondrial CYP11B2 (aldosterone synthase), which converts 11-deoxycorticosterone by 11β-hydroxylation and the formation of a carbon 18 aldehyde group toward aldosterone, is found only in the zona glomerulosa. The characteristic enzymes of the inner zones are the microsomal CYP17 (17α-hydroxylase/17,20 lyase) and the mitochondrial CYP11B1 (11β-hydroxylase). CYP17 catalyzes the 17α-hydroxylation of pregnenolone and progesterone as well as the side chain fission of 17α-hydroxy C21 steroids resulting in the formation of dehydroepiandrosterone (DHEA) or androstenedione (Fig. 19-2). CYP11B1 catalyzes the 11β-hydroxylation in the zona fasciculata and reticularis (Payne and Hales, 2004). The other steroidogenic enzymes are present in all three zones of the adrenal cortex (Müller, 1986). In rat, mouse, guinea pig, baboon, and hamster two distinct CYP11B forms are found, in contrast with cow, pig, sheep, and frogs where the formation of glucocorticoids and mineralocorticoids is catalyzed by a single 11β-hydroxylase (CYP11B1) (Lisurek and Bernhardt, 2004). The characteristic microsomal 21-hydroxylating (CYP21) and mitochondrial 11β-hydroxylating (CYP11B1) enzymes appear to have developed at an early stage of evolution and are present in all vertebrates. Also, the 18-oxygenated corticosteroids (CYP11B2) retain their importance as mineralocorticoids in all vertebrates. For the 17α hydroxylation (CYP17) potential, the situation is different. Most mammals secrete cortisol as the predominant glucocorticoid. However, rodents and birds secrete predominantly 17-deoxycorticosteroids such as corticosterone. In line with this, steroid determinations in adrenal venous blood of dogs (Hirose et al., 1977) have revealed cortisol/corticosterone ratios to range from about 3 to 7. In another study, ratios of secretion rates were found ranging from 1.2 to 2.7 and corticosterone/aldosterone secretion ratios between 7 and 25 (Dor et al., 1973). With HPLC analysis in peripheral blood of ACTH-stimulated dogs, the cortisol/corticosterone ratios ranged from 2.4 to 9.7 with a mean of 5.0 (Lothrop and Oliver, 1984). In addition to glucocorticoids and aldosterone, the adrenal vein blood of dogs and pigs contains androstenedione, 11-hydroxyandrostenedione, androsterone, pregnenolone, progesterone, and 11-hydroxyprogesterone (Heap et al., 1966; Holzbauer and Newport, 1969), as well as very small amounts of estradiol and estrone (Dor et al., 1973). FIGURE 19-2 Biosynthetic pathways for adrenal steroid production. Numbers by arrows denote specific enzymes: 1 = CYP11A1; 2 = CYP17; 3 = 3β-HSD; 4 = CYP21; 5 = CYP11B1; 6 = CYP11B2. In cats, the cortisol/corticosterone ratio has been estimated to range from 1.6 to 12.4, whereas in kittens the ratio is less than unity (Ilett and Lockett, 1969). In the peripheral blood of horses, higher cortisol/corticosterone ratios (16.0:0.5 and 7.0:0.5) have been reported (James et al., 1970; Zolovick et al., 1966). In birds, corticosterone, a glucocorticoid with minor mineralocorticoid properties, is by far the main adrenal secretory product. In the peripheral blood of adult birds, no cortisol could be demonstrated with radioimmunoassay (Walsh et al., 1985; Zenoble et al., 1985) or HPLC analysis (Lumeij et al., 1987). In chickens, the 17α-hydrolyase activity is present in embryonic life but decreases after hatching (Carsia et al., 1987; Nakamura et al., 1978). The steroidogenic activity of the two inner zones of the adrenal cortex is predominantly controlled by the pituitary hormone ACTH. The production of aldosterone in the zona glomerulosa is adapted to the sodium and potassium status of the organism by a complex, multifactorial, and mainly extrapituitary control system. For details on the secretion of ACTH by the pituitary and its regulation, refer to Chapter 18. In puppies, the feedback control of the hypothalamic-pituitary-adrenal axis is already operative at the time of birth (Muelheims et al., 1969), although up to the age of 8 weeks basal plasma cortisol concentrations are lower than in mature dogs. There is indirect evidence that this is related to low binding to transport proteins (Randolph et al., 1995). The action of ACTH on the adrenal gland is rapid; within minutes of its release, the concentration of steroids in the adrenal venous blood increases. The most likely mechanism by which ACTH stimulates steroidogenesis is via activation of membrane-bound adenylate cyclase. This increases the level of cyclic adenosine 3′,5′-monophosphate (cAMP), which activates adrenocortical protein kinases. This results in the phosphorylation of enzymes that enhance the rate of conversion of cholesterol to pregnenolone. Within the adrenal gland, a crosstalk exists with the adrenal medulla (Schinner and Bornstein, 2005). In vitro coculture of adrenocortical cells with chromaffin cells results in a 10-fold increase of steroidogenic activity (Haidan et al., 1998). Also, many intra-adrenal produced cytokines and growth factors play an important role in the regulation of steroidogenesis (Ehrhart-Bornstein et al., 1998). In adrenal tumors, a variety of ectopic and abnormal hormone receptors may stimulate adrenal corticoid release (Lacroix et al., 2001) (see also the section on adrenocortical diseases). In the regulation of aldosterone secretion, the two most important effectors are the peptide hormone angiotensin II (ANG II) and the extracellular potassium concentrations. Regulation of aldosterone secretion by the potassium status is direct and rather simple (Fig. 19-3). The second loop, which adapts aldosterone secretion to the sodium balance, is much more complex. These two systems are regulated by negative feedback loops. In contrast, ACTH is a representative of other factors that may stimulate aldosterone release without a link to negative feedback (Williams, 2005). From all factors known to regulate aldosterone production in vitro, the stimulation is probably confined under physiological conditions to stimulation by ANG II, K+, and ACTH and inhibition by the atrial natriuretic hormone (ANP) (Spat and Hunyady, 2004). FIGURE 19-3 Physiological control of aldosterone secretion (Müller, 1986). Apart from these factors, other intra-adrenal factors (Ehrhart-Bornstein et al., 1998) and environmental factors may regulate aldosterone production not only at the level of conversion of cholesterol to pregnenolone but also at the level of aldosterone synthase (CYP11B2) (Williams, 2005). At normal concentrations, only about 10% of the total blood cortisol and corticosterone is in the free form (i.e., susceptible to ultrafiltration). At body temperature, 70% of the plasma cortisol is bound to a globulin called transcortin or corticosteroid-binding globulin (CBG). Transcortin has a high affinity for cortisol and corticosterone, but its binding capacity is limited. Another 20% of plasma cortisol is bound to albumin although its affinity for cortisol is much less than that of transcortin. In line with these percentages, in the dog the free fraction has been estimated to range from 5% to 12% (Kemppainen et al., 1991; Meyer and Rothuizen, 1993). Transcortin is ubiquitous in mammals, but plasma concentrations vary considerably, resulting in species differences in total cortisol concentration. Most domestic animals have little corticosteroid-binding activity compared to humans (Rosner, 1969). As the free rather than the protein-bound steroid is biologically active, methods have been developed to measure free cortisol. By employing the combination of ultrafiltration and equilibrium dialysis, it was demonstrated that in dogs with portosystemic encephalopathy the associated hyperadrenocorticism is not only characterized by an increased total cortisol concentration in plasma but also by an increase in the free fraction of plasma cortisol (Meyer and Rothuizen, 1994). Between various pig breeds, a two-fold difference in plasma CBG binding capacity was found and related to increased drip loss in the Meishan breed (Geverink et al., 2006). The physiological significance of protein binding probably lies in a buffering effect, which prevents rapid variations of the plasma cortisol level. Transcortin restrains the active cortisol from reaching the target organ and also protects it from rapid inactivation by the liver and excretion through the kidneys. Plasma aldosterone is predominantly bound to albumin, which has a low affinity. The relatively low degree of protein binding of plasma aldosterone partially explains the very low plasma concentration and the short biological half-life of this hormone. Only unbound cortisol and its metabolites are filterable at the glomerulus. Most of this filtered cortisol is reabsorbed, whereby a tubular maximum is only achieved at very high filtered loads of free cortisol (Boonayathap and Marotta, 1974). Less than 20% of the filtered cortisol is excreted unchanged in the urine. Nevertheless, in most mammals the kidneys account for 50% to 80% of the excretion of the metabolized steroids. The remainder is lost via the gut. To render them suitable for renal elimination, the steroids are inactivated and made more water soluble through enzymatic modifications. The liver is the major organ responsible for steroid inactivation and conjugation to form water-soluble compounds, although in the dog—contrary to humans—the kidney and the gastrointestinal tract also contribute to the metabolic clearance of cortisol (McCormick et al., 1974). In the canine, kidney cortisol glucuronide is both secreted and reabsorbed, without a tubular maximum or a plasma threshold (Boonayathap and Marotta, 1974). Cortisol is cleared from the plasma with a half-life of 60 min or less. For pigs, the metabolic clearance rate of cortisol was calculated to be about 1l.h–1.kg–1 (Hennesy et al., 1986). In dogs, about 60% of infused cortisol is eliminated within 24 h in the urine (Rijnberk et al., 1968a). The 11β-hydroxyl group of cortisol can be oxidized to the ketone, forming cortisone (Fig. 19-4). The reaction is reversible, and in general the equilibrium is shifted to favor the 11β-hydroxyl group. However, because the adrenal cortex produces much more cortisol than cortisone (if any), there is substantial cortisol-to-cortisone conversion. These two steroids have similar subsequent metabolic fates. FIGURE 19-4 Metabolism of cortisol in the dog (simplified). Apart from this 11β-hydroxylation, cortisol metabolism in the dog involves the following: (1) reduction of ring A to tetrahydro derivatives, (2) reduction of the 20-keto group to a hydroxyl, and (3) conjugation with glucuronic acid to form glucuronides (Gold, 1961). In addition, unconjugated 20-hydroxycortisol/cortisone has been found in canine urine. In total glucuronide fraction of urinary corticoids in dogs, at least steroids reduced at C-20 represent 60%. This is of prime importance when it comes to assessing adrenocortical function by measuring urinary cortisol metabolites. Measurements directed at steroids containing a 17α,21-dihydroxy, 20-keto arrangement (i.e., the Porter-Silber reaction) will detect only a small part of the cortisol metabolites and therefore have limited value (Siegel, 1965). Instead, preferably, measurements are preformed involving reduction of the urine metabolites at C-20, followed by oxidation to 17-ketosteroids, which are then quantitated. Further details on this measurement of total 17-hydroxycorticosteroids are given in Section IV.B.3. Aldosterone is converted not only to tetrahydroaldosterone-3-glucuronide but also to aldosterone-18-glucuronide. Most of aldosterone metabolism takes place in liver and kidney, but the intestine and spleen might also contribute to a minor degree (Balikian, 1971). In domestic animals, the catabolism of androgens has not been studied in any detail. For the dog, it is known that little of the secreted androgens can be measured as 17-ketosteroids in urine (Rijnberk et al., 1968b; Siegel, 1967). Probably most of the excretion occurs via the bile. In the cat, glucocorticoid excretion is also largely biliary (Rivas and Borrell, 1971; Taylor, 1971). Feline liver function has several specific features, including a relatively low glucuronyl-transferase activity. Hence, formation of glucuronide conjugates is low, and the conjugates are mainly sulphates, which are mostly excreted via the biliary route. Despite this low urinary excretion of metabolites and conjugates, it was shown that renal excretion of free cortisol is sufficient for diagnostic purposes (Goossens et al., 1995) (see also Section IV.B). Apart from the use of urinary excretion to examine the glucocorticoid status, fecal glucocorticoid metabolite measures are increasingly being used, especially for zoo and wildlife animals. Interpretation of fecal glucocorticoids may be confounded by a large variety of factors that should be taken into account (Millspaugh and Washburn, 2004). Of the naturally occurring glucocorticoids (cortisol, cortisone, and corticosterone), cortisol is the most potent. Several of the synthetic analogues of cortisol are more potent than cortisol itself (Table 19-1). Figure 19-5 illustrates the structural features that determine glucocorticoid potency. FIGURE 19-5 Structure-function relationships of corticosteroids. The bold lines and letters indicate the structure common to all glucocorticoids. Substituents that may enhance glucocorticoid activity are presented by light lines and letters. In their target organ cells, glucocorticoids act in the same manner as other steroid hormones: diffusion through the cell membrane; binding to the cytoplasmic, glucocorticoid (GR), and mineralocorticoid (MR) receptors; translocation of the hormone receptor complex to the nucleus; and subsequent stimulation of the synthesis of specific RNAs leading to synthesis of specific enzymes. The latter include key enzymes in gluconeogenesis such as fructose-1,6-disphosphatase, glucose-6-phosphatase, and pyruvate carboxylase. In the dog, corticosteroid excess also results in induction of an isoenzyme of alkaline phosphatase. This corticosteroid-induced form of alkaline phosphatase has not yet been found in other species. The marked stability at 65°C allows easy quantitation in plasma as a diagnostic screening tool for cases of suspected hyperadrenocorticism (Teske et al., 1986, 1989). The overall effect of glucocorticoids on metabolism is to supply glucose to the organism by the transformation of proteins. This occurs via the above-mentioned induction of gluconeogenic enzymes in the liver. Thus, glucocorticoids divert metabolism from a phase of growth and storage toward increased physical activity and energy consumption, whereas chronic excess leads to catabolic effects such as muscle wasting, skin atrophy, and osteoporosis. The tendency to hyperglycemia is opposed by increased secretion of insulin, which in turn tends to enhance fat synthesis. This along with the increased food intake owing to central appetite stimulation (Debons et al., 1986) explains the (centripetal) fat deposition, manifested by abdominal enlargement. In situations of glucocorticoid deficiency, water excretion is impaired, whereas glucocorticoid excess may result in polyuria, being most pronounced in the dog. This is in part due to antagonism of cortisol to the action of vasopressin. In addition, glucocorticoid excess causes loss of the sensitivity of the osmoregulation of vasopressin release (Biewenga et al., 1991). Even physiological increases in cortisol may inhibit basal vasopressin release in dogs (Papanek and Raff, 1994). Glucocorticoids have long been known to have effects on blood cells, including a reduction in the numbers of eosinophils and lymphocytes and an increase in the number of neutrophils and hence in the total number of leukocytes. Glucocorticoid deficiency leads to normochromic, normocytic anemia. As far as effects on other endocrine glands are concerned, it is shown that canine hyperadrenocorticism results in reversible suppression of growth hormone secretion (Peterson and Altszuler, 1981). The frequently observed lowering of circulating thyroxine concentrations has been ascribed to changes in the thyroid hormone binding capacity of the plasma and to inhibition of lysosomal hydrolysis of colloid in the thyroid follicular cell (Kemppainen et al., 1983; Woltz et al., 1983). Glucocorticoids have multiple effects on peripheral transfer, distribution, and metabolism (Kaptein et al., 1992), but thyroid function does not seem to be affected (Rijnberk, 1996). The glucocorticoid prednisone was found to inhibit LH secretion in male dogs, leading to reduced testosterone concentrations (Kemppainen et al., 1983). In health, adrenocortical production of androgens is trivial in comparison with the production of these hormones by the gonads. However, a pathological excess of adrenal androgens might induce virilization (i.e., the development of masculine secondary sex characteristics), which would be most noticeable in the female or immature male. So far, no virilization as a consequence of enzyme deficiency has been reported in domestic animals, but in equine hyper-adrenocorticism, hirsutism is a common feature (Pauli et al., 1974; Van der Kolk et al., 1993). The widespread MR has equal affinity for aldosterone and the glucocorticoids cortisol and corticosterone, whereas the latter two hormones circulate at much higher concentrations than aldosterone. However, in the classical aldosterone targets (kidney, colon, and salivary gland), the enzyme 11β-hydroxysteroid dehydrogenase (11βHSD) converts cortisol and corticosterone (but not aldosterone!) to the 11-keto analogues. These analogues cannot bind to MR, thereby enabling aldosterone to occupy this receptor (Funder, 2005). Thus, not the receptor but a prereceptor modification provides the tissue specificity. The synthetic steroid 9α-fluorocortisone (Table 19-1) binds tightly to mineralocorticoid receptors and is used for mineralocorticoid replacement therapy because it is more stable than aldosterone after oral administration. Mineralocorticoid antagonists such as spironolactone bind to these receptors and in this way block aldosterone action. Aldosterone controls the volume and the cationic composition of the extracellular fluid by regulating sodium and potassium balance. Its main action is on the tubular apparatus of the kidneys, but aldosterone receptors are also found in the gut, the salivary glands, and the sweat glands. In the kidney, the effect of aldosterone on the distal tubule consists almost entirely of an exchange of sodium with potassium and hydrogen ions. Aldosterone also promotes the excretion of magnesium and ammonium ions. In the absence of extraordinary stress, the plasma cortisol concentration of healthy animals varies within certain limits, although adrenocortical secretion does not occur evenly throughout the day but rather in bursts. In humans, most of the secretory bursts occur between midnight and early morning, leading to a diurnal rhythm of circulating cortisol levels. The temporal coincidence between secretory bursts and plasma ACTH concentrations indicates neuroendocrine control. In domestic animals and certainly in the dog, initially there has been some controversy as to the occurrence of circadian variation in cortisol concentrations. Later definite evidence for episodic but not circadian fluctuations in plasma cortisol concentrations in dogs was presented (Kemppainen and Sartin, 1984a). In the horse (Dybdal et al., 1994) and the pig (Benson et al., 1986; Bottoms et al., 1972; Janssens et al., 1995b; Larsson et al., 1979) as well as in sheep (Fulkerson and Tang, 1979), bulls (Thun et al., 1981), pigeons (Joseph and Meier, 1973; Westerhof et al., 1994), and chickens (Lauber et al., 1987), the occurrence of circadian variations is generally acknowledged. Also in the cat, there have been reports on the occurrence of an (opposite) diurnal rhythm. However, in later reports this was not confirmed (Johnston and Mather, 1979; Leyva et al., 1984). As in the dog, the secretion is episodic without evidence for a diurnal rhythm (Peterson and Randolph, 1989). Apart from relatively short-term changes such as episodic secretion and diurnal variation, plasma cortisol concentration may change with age and during the estrus cycle, during pregnancy, and around the time of parturition. For example, basal cortisol concentrations in puppies 8 weeks of age or younger are lower than in mature dogs, most probably because of reduced binding to plasma proteins rather than to altered secretion patterns (Randolph et al., 1995). In gilts a discrete cortisol peak occurs during the early follicular phase of the sexual cycle, coinciding with the decline in plasma progesterone levels (i.e., at 4 days before the plasma LH surge) (Janssens et al., 1995c). Plasma cortisol concentrations of pregnant cows decrease significantly during the fourth month of pregnancy. Thereafter they remain fairly constant until the fifth and sixth day before parturition, when a sharp rise is seen, and also at around 24 h before parturition plasma corticoids increase again (Eissa and El-Belely, 1990). A highly significant increase in basal plasma cortisol concentrations occurs with aging in dogs (Goy-Thollot et al., 2006) with no differences in ACTH-stimulated plasma cortisol concentrations. This confirms earlier studies (Rothuizen et al., 1993) where the increased basal activity of the hypothalamo-pituitary-adrenal axis is attributed to a decrease in limbic type-I MR concentrations, whereby no differences were found for the type II GR in canine brain structures. The increase in pituitary type II GR with aging explains the intact feedback in the aging dog (Rothuizen et al., 1993). The ACTH-stimulated increase in plasma aldosterone concentrations was found to be significantly reduced with aging (Goy-Thollot et al., 2006). Independent of these physiological variations, it is clear that stress may activate the pituitary-adrenocortical system. Factors such as housing (Carlstead et al., 1993; Cockram et al., 1994; Koelkebeck and Cain, 1984), lactation (Gwazdauskas et al., 1986), exercise (Dybdal et al., 1980; Foss et al., 1971; Rossdale et al., 1982; Sloet van Oldruitenborgh-Oosterbaan et al., 2006), surgery (Robertson et al., 1994), anaesthesia, heat (Gould and Siegel, 1985), emotional strain (Bobek et al., 1986; James et al., 1970; Kirkpatrick et al., 1977), food deprivation (Messer et al., 1995), and anticipation of feeding (Murayama et al., 1986) all lead to increased corticosteroid secretion. In dogs, a visit to a veterinary practice, orthopedic examination, and hospitalization increased the urinary corticoid:creatinine ratio, which is a measure for adrenocortical function (Van Vonderen et al., 1998). In the horse, during exercise increased plasma renin activity results in a considerable rise in aldosterone secretion (Guthrie et al., 1980, 1982). From the lack of any increase in plasma corticosterone in homing pigeons during flight, it was concluded that the animals were not under serious stress (Viswanathan et al., 1987). With chronic stress, such as during prolonged tethered housing of pigs, an increased responsiveness of the adrenal cortex to ACTH is induced, whereas the sensitivity of the pituitary to stimulation with corticotrophin releasing hormone (CRH) or vasopressin remains unaltered, and the challenge (nose sling)-induced ACTH response is lower than in loosely housed pigs. This indicates that during chronic stress, mitigating mechanisms become operational, most probably mediated by endogenous opioids (Janssens et al., 1995a). According to Selye’s theory of general adaptation, corticosteroids are needed for the (metabolic and circulatory) defense reaction. Administration of cortisol to improve nonspecific resistance is indicated in established pituitary or adrenal insufficiency. From an immunological point of view, stress suppresses defense reactions. Stress-induced glucocorticoid secretion prevents the overshooting of the animal’s reactions to stress. The glucocorticoids modulate the mediators of the immune system such as the different lymphokines and mediators of the inflammatory reactions: prostaglandins, leukotrienes, kinins, serotonin, and histamine (Munck et al., 1984). It is now well established that the interaction of the neuroendocrine system and the immune system is not a one-way but a bidirectional communication. Tissue injury or inflammation elicits production of immunoregulatory cytokines (lymphokines and monokines) by macrophages and monocytes. These cytokines also activate the pituitary-adrenal axis and increase glucocorticoid concentrations, whereas the production and action of these immune mediators are inhibited by glucocorticoids. Thus, there is strong evidence for the existence of a feedback circuit, in which immunoregulatory cytokines act as afferent and ACTH and glucocorticoids as efferent hormonal signals (Besedovsky et al., 1986; Woloski et al., 1985). The regulatory actions of the cytokines are exerted at the level of the hypothalamus, where CRH is the major mediator of the response (Berkenbosch et al., 1987), although cytokines also exert an influence at the level of the pituitary and the adrenals (Gaillard, 1994). As this chapter is not meant to cope with clinical and pathological details, discussion of adrenocortical diseases is confined to definitions, short statements on occurrence in species, and referral to key references. In textbooks on endocrine diseases of companion animals (Drazner, 1994; Feldman and Nelson, 2004; Rijnberk, 1996), the adrenocortical diseases of the dog and the cat are described in detail. Adrenocortical hypofunction includes all conditions in which the secretion of adrenal steroid hormones falls below the requirements of the animal. It may be divided into two categories: 1. Primary hypoadrenocorticism or Addison’s disease is the result of deficiency of both glucocorticoid and mineralocorticoid secretion from the adrenal cortices (primary adrenocortical failure). “Idiopathic” or immune-mediated atrophy (Schaer et al., 1986) of all zones is the most frequently observed histopathological lesion, although the lymphocytic adrenalitis may be confined to the zona fasciculata and zona reticularis (Kooistra et al., 1995). It is well known in the dog and rare in the cat (Peterson and Randolph, 1989). 2. Secondary hypoadrenocorticism is the result of pituitary ACTH deficiency, causing decreased glucocorticoid secretion. Because of the almost unaltered aldosterone secretion, it will usually remain unnoticed. It is observed occasionally in dogs with pituitary insufficiency resulting from pituitary cystic lesions leading to an empty sella or as a result of a compressive nonfunctioning (endocrine inactive) pituitary macroadenoma. Animals receiving long-term corticosteroid treatment, despite physical and biochemical hyperadrenocorticoid changes, develop secondary adrenocortical insufficiency because of prolonged hypothalamo-pituitary suppression. Atrophy of the two inner zones of the adrenal cortex results from the loss of endogenous ACTH stimulation. This is observed in the species in which corticosteroid therapy is common practice such as the dog (Greco and Behrend, 1995; Rijnberk, 1996) and the horse (Hoffsis and Murdock, 1970; Toutain and Brandon, 1983). It has also been observed in dogs and cats treated with progestagens, owing to the glucocorticoid activity that is intrinsic to these drugs (Chastain et al., 1981; Mansfield et al., 1986; Middleton et al., 1987; Selman et al., 1994). In principle, the adrenal cortex of animals might, as in humans, give rise to three distinct clinical syndromes of hyperfunction: mineralocorticoid excess or hyperaldosteronism (Conn’s syndrome), glucocorticoid excess or hypercortisolism (Cushing’s syndrome), and androgen excess (adrenogenital syndrome). Hypercortisolism is common in the dog though rare in the cat and the horse. In about 15% of hyperadrenocorticoid dogs, the disease is due to a primary adrenocortical tumor (ACTH independent). The excessive secretion of cortisol by these tumors may either be autonomous or associated with aberrant expression of ectopic or overexpression of eutopic adrenocortical receptors (Lacroix et al., 2001). For example, the expression of ectopic receptors for gastric inhibitory polypeptide may result in “food-dependent hypercortisolism.” In the other 85% of cases, the (ACTH-dependent) hypercortisolism is associated with a pituitary lesion, producing excess ACTH. The pituitary corticotroph adenomas may reside in either the anterior lobe or the intermediate lobe (Kemppainen and Peterson, 1994; Peterson et al., 1982). In rare cases, Cushing’s syndrome may also be due to ectopic secretion of ACTH by a neuroendocrine tumor outside the pituitary gland (Galac et al., 2005). In cats, hypercortisolism (Cushing’s syndrome) is usually concurrent with diabetes mellitus and may be either primary adrenocortical or pituitary dependent (Meij et al., 2001, 2004; Meijer et al., 1978b; Peterson and Randolph, 1989). Primary hyperaldosteronism in cats may be due to an adrenocortical tumor (Ash et al., 2005; Flood et al., 1999; Mackay et al., 1999; Moore et al., 2000; Rijnberk et al., 2001) or to bilateral hyperplasia of zona glomerulosa tissue (Javadi et al., 2005). Prominent clinical features in cats with hyperaldosteronism are hypokalemia, episodic weakness, cervical ventroflexion, arterial hypertension, and vision loss resulting from retinal detachment. The nontumorous form of primary hyperaldosteronism in cats is associated with progressive renal disease (Javadi et al., 2005). In the horse, hypercortisolism (Cushing’s syndrome) originates almost invariably in the intermediate lobe of the pituitary (Orth et al., 1982). In domestic ferrets, hyper-adrenocorticism is quite common and it is primarily associated with adrenocortical tumor or nodular adrenocortical hyperplasia (Rosenthal et al., 1993). Domestic ferrets with hyperadrenocorticism have no detectable abnormalities in plasma concentrations of ACTH or α-MSH (Schoemaker et al., 2002a) and increased urinary corticoid/creatinine ratios resistant to dexamethasone suppression (Schoemaker et al., 2004), both indicating ACTH-independent hyperadrenocorticism. Positive staining was found with the LH receptor antibody in hyperplastic or neoplastic adrenal glands of hyperadrenocorticoid ferrets supporting the hypothesis that gonadotropic hormones play a role in the pathogenesis of hyperadrenocorticism in ferrets (Schoemaker et al., 2002b). In both hypoadrenocorticism and hyperadrenocorticism, there are a number of abnormal laboratory findings that are more common than in other diseases. Many of these abnormalities can be derived from the above-described action of glucocorticoids and mineralocorticoids (Section II.F). The essentials are summarized here. In primary hypoadrenocorticism, the decreased aldosterone production results in hyponatremia and hyperkalemia. The associated hypovolemia leads to prerenal uremia. In hyperadrenocorticism, the classic hematological abnormality is eosinopenia, which may be associated with lymphopenia and occasionally with leukocytosis and erythrocytosis. A common biochemical abnormality is the elevated plasma concentration of alkaline phosphatase (Eckersall and Nash, 1983) that may occur in conjunction with mild elevation of alanine aminotransferase (ALT). In addition, hyperglycemia, hyperlipidemia, and low thyroxine concentrations may be observed. Urine-specific gravity is usually low, and in 5% to 10% of cases glucosuria is found. Of these abnormalities, the elevated alkaline phosphatase (AP) concentration is the most common laboratory abnormality in dogs with corticosteroid excess (either exogenous or endogenous). This increase is due to the induction of a specific isoenzyme, which has greater heat stability at 65°C than other AP-isoenzymes (Teske et al., 1986) and is therefore easily measured by a routine laboratory procedure. An abnormally elevated AP-65°C value may point to hyperadrenocorticism, but it is unsuitable as a diagnostic test because of its low specificity (Teske et al., 1989). The low thyroxine (T4) concentrations in plasma, which may be observed in hyperadrenocorticism, seem to be a consequence of altered transport, distribution, and metabolism of T4 rather than the result of hyposecretion (Rijnberk, 1996). There may be other conditions causing these abnormalities, however, and some cases do not present with typical routine laboratory characteristics. Therefore, a detailed understanding of the specific laboratory tests commonly used to investigate adrenocortical function is essential. In addition, the spontaneous forms of hyperadrenocorticism may arise from pituitary ACTH overproduction or from autonomously hypersecreting adrenocortical tumors. Each of these requires a different mode of treatment, and therefore insight into the tests used in the differential diagnosis is of prime importance. The availability of highly specific radioimmunoassays has greatly enhanced and simplified assessment of adrenocortical function. Some of the older (chemical) methods, which are now seldom used, are mentioned briefly. Methods in common use are described in more detail, which applies especially to the dynamic tests, part of which is used in the differential diagnosis (Section IV.C). In the radionuclide dilution method, the rate of cortisol production is determined through administration of radiolabeled cortisol and isolation of cortisol metabolites from urine that is collected over at least 24h. The specific activities of labeled cortisol and tetrahydrocortisol (THF) or tetrahydrocortisone (THE) are compared. Some preliminary work (Rijnberk et al., 1968b) indicated that this test worked for the dog and might potentially be very useful. However, the laborious character of the procedure rules out general availability. Plasma corticoid concentrations are subject to considerable variation owing to the pulsatile nature of the secretion, physiological variation (see Section II.G), and alterations in transport proteins (Meyer and Rothuizen, 1994). Therefore, single determinations are regarded of little diagnostic value in assessing hypo- or hyperadrenocorticism. Nevertheless, in a considerable percentage of cases, resting values outside the reference ranges may be found. It has been demonstrated recently that calculation of the ratio of plasma cortisol:ACTH concentrations in hypoadrenocorticism did not show an overlap with values of healthy control dogs (Javadi et al., 2006). There are few data on sex and breed differences, and they are not dealt with in Table 19-2. Nevertheless there is evidence that they should be taken into account (Frank et al., 2003a). In dogs, circulating cortisol concentrations do not differ between males and females, but in small dogs higher values are found than in large breed dogs (Reimers et al., 1990), and neutered males may have lower plasma cortisol concentrations in comparison to intact males (Frank et al., 2003b). Also in pigs, breed-specific basal plasma cortisol concentrations exist (Sutherland et al., 2006). With measurements of urinary corticoids or their metabolites, an integrated reflection of corticoid production over a period of time is obtained, thereby adjusting for the fluctuations in the plasma levels. Indeed, in dogs measurements of 17-hydroxycorticosteroids in 24-hour urine samples were found to have a high discriminatory power (Table 19-3; Rijnberk et al., 1968a). However, the method has been abandoned because of the complicated and time-consuming chemical analysis, in combination with the difficulty of accurate collection of 24-hour urine samples.
I. ANATOMICAL CONSIDERATIONS
II. PHYSIOLOGY OF ADRENOCORTICAL HORMONES
A. Steroid Nomenclature
B. Biosynthesis
C. Regulation of Secretion
D. Transport
E. Metabolic Breakdown and Excretion
F. Steroid Receptors and Actions
1. Glucocorticoids
2. Adrenal Androgens
3. Mineralocorticoids
G. Physiological Variation, Stress, and the Immune System
III. ADRENOCORTICAL DISEASES
A. Hypoadrenocorticism
B. Hyperadrenocorticism
IV. ASSESSMENT OF ADRENOCORTICAL FUNCTION
A. Routine Laboratory Diagnostics
B. Tests of Basal Adrenocortical Function
1. Cortisol Production Rate
2. Plasma Corticoids
3. Urinary Corticoids
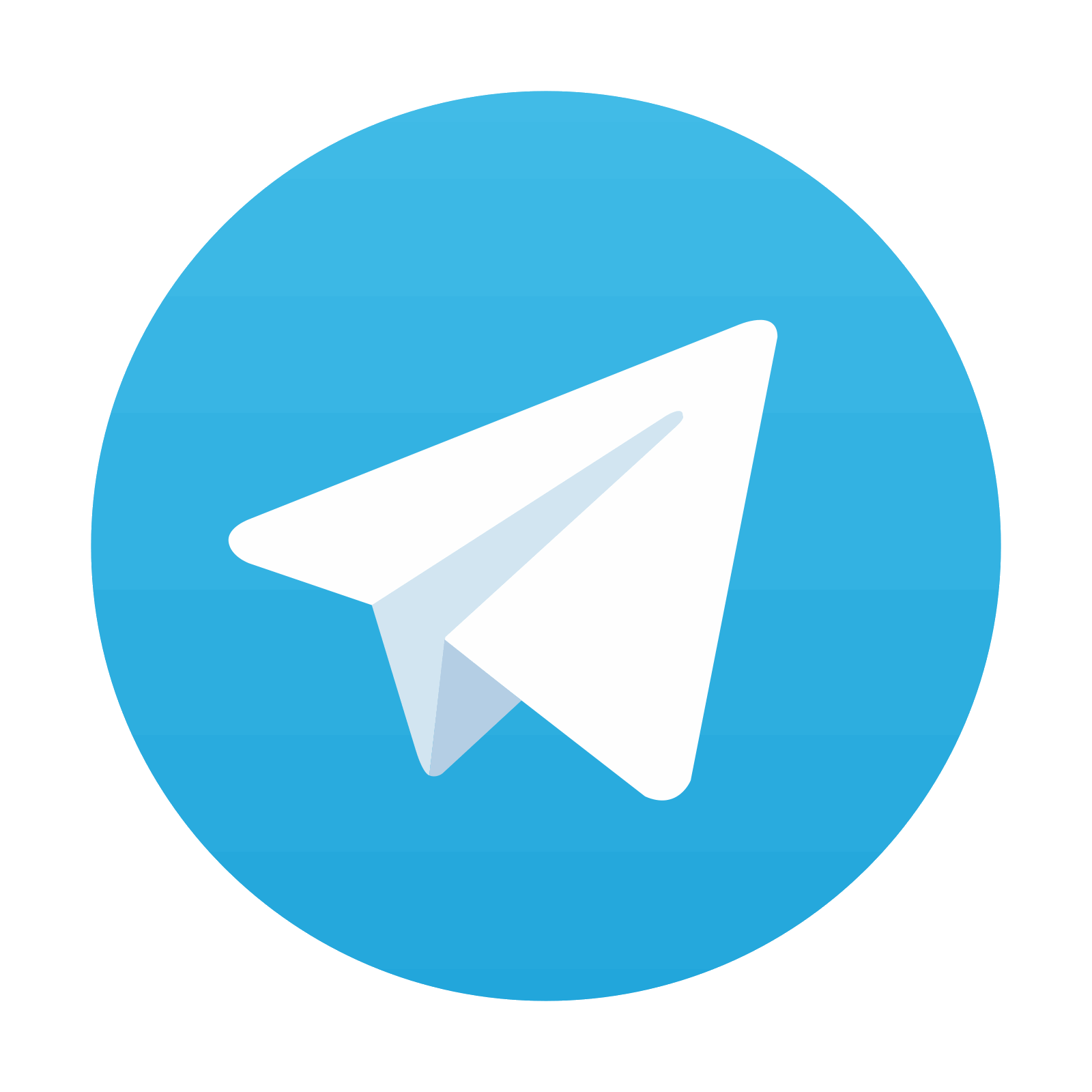
Stay updated, free articles. Join our Telegram channel
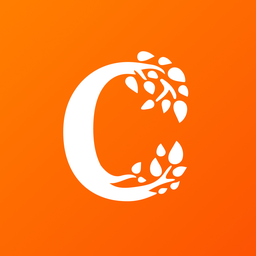
Full access? Get Clinical Tree
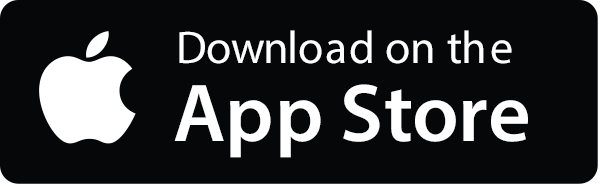
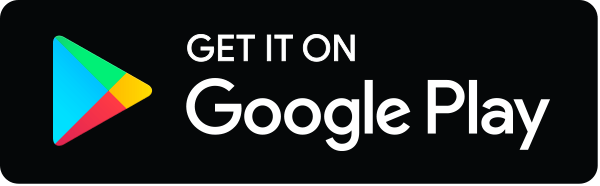