Janine T. Bossé Adina R. Bujold and Lu Li Members of the genus Actinobacillus are small, non‐flagellated, Gram‐negative, pleomorphic, coccobacillary rods. They are facultatively anaerobic, benefiting from addition of 5% CO2 for optimal growth at 37°C. All species have complex nutritional requirements, but most, apart from Actinobacillus pleuropneumoniae and some Actinobacillus suis isolates will grow on MacConkey’s agar as tiny lactose‐fermenting colonies. Characteristic phenotypes of the genus include positive urease and β‐galactosidase activities, negative indole reaction, and reduction of nitrates. Actinobacilli are associated with mucous membranes of animals, typically with limited host range. Many species infect farm animals, whereas Actinobacillus hominis and Actinobacillus ureae are opportunistic pathogens of man. Species classification based on phenotypes such as nutritional requirements, or by serological typing, has resulted in some misclassifications. Sequence‐based phylogenies, especially using multiple genes or whole genomes, are helping clarify the taxonomy of Pasteurellaceae species, though many remain to be formally reclassified. There are currently 10 species within the genus Actinobacillus sensu stricto (Blackall and Turni 2020), eight of which infect animals other than humans, including the recently identified Actinobacillus vicugnae, isolated from alpaca. Other Actinobacillus species with valid names standing in nomenclature (https://lpsn.dsmz.de/genus/actinobacillus) are not Actinobacillus sensu stricto but have yet to be reclassified. Recent whole‐genome sequencing supports recognition of the non‐pathogenic swine commensal “[Actinobacillus] porcitonsillarum” as a novel species (Donà and Perreten 2018). It is phenotypically very similar to A. pleuropneumoniae, but it is most closely related to [Actinobacillus] minor. A summary of important Actinobacillus and Actinobacillus‐like species found in animals is presented in Table 12.1 and those of particular interest are discussed in more detail in subsequent sections. Animals belonging to the species Sus scrofa (i.e. wild boar, domestic and feral pigs) are the natural hosts of A. pleuropneumoniae. Wild boar populations in Europe and North America carry A. pleuropneumoniae, but it is not known whether they are a source of infection for commercial swine (Sassu et al. 2018). Reports of A. pleuropneumoniae isolation from chickens (Pérez Márquez et al. 2014) and horses (Layman et al. 2014; Uchida‐Fujii et al. 2019) lack confirmation. Two recent horse isolates were positive for the A. pleuropneumoniae species‐specific apxIV gene by polymerase chain reaction (PCR), but the 16S rRNA sequences showed highest identity (98.6–98.9%) with Actinobacillus lignieresii (Uchida‐Fujii et al. 2019). Table 12.1 Actinobacillus sensu stricto and Actinobacillus‐like species isolated from animals. a) Actinobacillus‐like species, not belonging to Actinobacillus sensu stricto, are shown with square brackets around the abbreviated genus designation, i.e. [A.]. b) Most Actinobacillus and Actinobacillus‐like species are commensals of the alimentary, respiratory, or urogenital tract, but some are opportunistic pathogens causing sporadic cases of the diseases shown. Only A. pleuropneumoniae is considered a primary pathogen, though may behave as a commensal. c) Equine strains previously classified as A. lignieresii, that are genetically most closely related to A. equuli subsp. haemolyticus, have been designated Actinobacillus genomospecies 1. d) Sorbitol negative isolates of A. arthritidis have been designated as Actinobacillus genomospecies 2. Pleuropneumonia can occur in pigs of all ages, with the source of infection originating outside or within a herd (Figure 12.1). Mixing infected with uninfected pigs, or those carrying different serovars, can precipitate disease. However, in herds with many asymptomatic animals, outbreaks of disease may be very rapid suggesting environmental triggers (including stress and/or coinfection with other bacteria or viruses) may play a significant role in transmission (Klinkenberg et al. 2014). A. pleuropneumoniae is considered a parasite of the porcine respiratory tract, mainly found in the tonsils of asymptomatic carriers, and most large swine production units are endemically infected (Sassu et al. 2018). More than one serovar can be found in a herd (even in individual pigs) and serological testing of subclinically infected animals may reveal the presence of serovar(s) that are different from those recovered from clinical lung samples from the same herd. Vertical transmission from infected sows to some piglets prior to weaning is followed by horizontal transfer to a larger number of piglets post‐weaning when maternal antibodies are depleted (Tobias et al. 2014). A. pleuropneumoniae is transmitted by direct pig‐to‐pig contact or aerosols. Poor survival outside of the host, especially in hot/dry climates, may be mitigated by the presence of mucus or other organic matter (Assavacheep and Rycroft 2013). Detection of A. pleuropneumoniae in biofilms from drinking taps on pig farms in Mexico was reported (Loera‐Muro et al., 2013), with incorporation into multispecies biofilms suggested to provide key nutrients such as NAD (Loera‐Muro et al., 2016; Wang et al., 2020), normally lacking outside the host. Figure 12.1 Overview of A. pleuropneumoniae (APP) transmission and development of disease. Adapted with permission from Sassu et al. (2018). See main text for further details. A. pleuropneumoniae isolates can be differentiated into two biovars, with the less prevalent biovar 2 isolates carrying an intact nadV gene that enables synthesis of nicotinamide adenine dinucleotide (NAD) from specific pyridine nucleotides or their precursors. Biovar 1 isolates have a truncated nadV and require exogenous NAD for growth. Although some serovars may be either biovar, serovars 1–12, and 15–19 are generally biovar 1; whereas most serovar 13 and 14 isolates are biovar 2 (Table 12.2). Identification of A. pleuropneumoniae serovars has traditionally been performed using serological methods that employ specific antisera for detection of surface polysaccharide antigens. Specificity has been ascribed to capsular polysaccharide (CPS or K antigen), whereas common lipopolysaccharide (LPS) O‐chain antigens (O‐Ags) present in subsets of serovars (1/9/11; 3/6/8/15/17/19; 4/7/18) may contribute to serological cross‐reactivity. In addition to cross‐reactivity issues, difficulties in standardizing sets of reference antisera have resulted in a shift to molecular typing methods based on detection of specific CPS biosynthesis (cps) genes. With the recent identification of serovar 19 (Stringer et al. 2021), a set of two multiplex PCRs has been described for typing of all known serovars. Table 12.2 Properties and distribution of A. pleuropneumoniae serovars. a) cps type refers to genetic classification of the capsule biosynthetic loci (Bossé et al. 2018). b) The O‐Ag/groups indicated are those typically associated with the specified serovars. Some exceptions have been described (Sassu et al. 2018). c) The Apx toxin profiles indicated are those typically associated with the specified serovars. Some exceptions have been described (Sassu et al. 2018). Based on common core genes, there are four types of cps loci in A. pleuropneumoniae. Type I cps loci (in serovars 2, 3, 6–9, 11, 13, and 17) produce teichoic acid polymers joined with phosphodiester linkages; type II loci (in serovars 1, 4, 12, 14, 15, 18, 19) produce repeating oligosaccharide polymers with phosphate linkages; and type III loci (in serovars 5 and 10) produce glycosidically linked oligosaccharides. The serovar 16 CPS structure has not yet been determined and the locus has been designated as type IV (Bossé et al. 2018). Although subtypes (a and b) of serovars 1 and 5 have been described based on minor differences in the CPS structure, these are not discriminated by molecular typing PCRs. Also, despite separate designations for serovars 9 and 11, they have very similar CPS and identical O—Ag structures, making them serologically highly cross‐reactive. Furthermore, with only a single bp difference in their cps loci, they cannot be differentiated by PCR and can be considered a hybrid 9/11 serovar (Stringer et al. 2021). Within serovars, isolates of A. pleuropneumoniae are fairly clonal and specific K antigens are normally associated with particular O‐Ags (Table 12.2), although exceptions have been described (Gottschalk and Broes 2019; Stringer et al. 2021). Apx toxin profiles also tend to be serovar‐specific, although, again, exceptions have been reported, most notably between different biovar isolates of the same serovar (Sassu et al. 2018). Diagnostic multiplex PCRs detecting serovar‐specific cps sequences will not identify isolates with atypical O‐Ag or Apx toxin genes. As the Apx toxin profile is related to virulence, an mPCR for detection of genes required for production and secretion of ApxI–III (see Section Damage to host tissues, below) can be run alongside serovar typing multiplex PCRs. Diagnostic typing is important for epidemiological tracking of disease‐related isolates as changes in geographic distribution over time may indicate emergence of new serovars or local changes in host/pathogen dynamics affecting disease control. Moreover, detailed knowledge of serovar prevalence and related Apx toxin profiles informs choice of vaccination strategy, but in many countries these data are not available. Establishment of an international epidemiological database has been recommended (Sassu et al. 2018) to track these details, together with relevant clinical metadata and antimicrobial resistance profiles, but this has not yet been implemented. Pleuropneumonia can range from peracute to chronic, depending on the immune status of the host, as well as concentration and serovar of bacteria reaching the lung. During peracute/acute disease, pigs may exhibit some/all of the following clinical signs: high fever, increased respiratory rate, coughing, sneezing, dyspnea, anorexia, ataxia, vomiting, diarrhea, and severe respiratory distress with cyanosis. Lung infections during acute/peracute disease, characterized by severe edema, inflammation, hemorrhage, and necrosis, are associated with increased numbers of the bacterium in lung exudates and nasal secretions, facilitating transmission. Histologically, marked polymorphonuclear leukocyte (PMN) infiltration, edema, and fibrinous exudate are apparent in the early stages of lung infection. As the disease progresses, macrophage infiltration increases, dense bands of degenerating leukocytes surround necrotic foci, and severe necrotizing vasculitis leads to hemorrhage in the lung. A. pleuropneumoniae can be found within alveolar and interlobular fluid and may spread from the parenchyma to the pleura via lymph vessels. The thoracic cavity is often filled with serosanguinous fluid and fibrin clots, and diffuse fibrinous pleuritis and pericarditis are common. Although systemic disease is rarely reported, recent studies have indicated multiorgan spreading may be common when lung infection is extensive (Hoeltig et al. 2018a). Complete resolution of lesions may occur upon recovery from acute pleuropneumonia. Alternatively, focal necrotic sequestra and/or well‐encapsulated abscesses, as well as fibrinous pleuritis, may persist. The pathogenesis of acute pleuropneumonia involves colonization of the respiratory tract mucosa, evasion of host clearance mechanisms, and damage of variable severity to host tissues. Over the past decade, advances in genomic and transcriptomic analysis techniques have markedly increased our understanding of both pathogen virulence factors and host factors involved in each stage, as discussed in detail below. An overview of key elements in pathogenesis is shown in Figure 12.2. Figure 12.2 Overview of A. pleuropneumoniae pathogenesis. Although adherence to host cells and nutrient acquisition are both part of colonization, they are shown separately here to indicate the various bacterial components which contribute to these functions. Conversely, evasion of host defenses and damage to host, although discussed as separate stages of pathogenesis in the main text, are shown together here, as Apx toxins and lipopolysaccharide (LPS) contribute to both functions, as can TolC. Although shown here as part of the Apx toxin secretion system, TolC (of which there are two orthologues in A. pleuropneumoniae) is also involved in efflux of toxic compounds. Some bacterial components (e.g. LPS) have been implicated in all of the stages, while other less obvious overlaps also exist, for example type 4 pili (Tfp), are important in adherence and also in uptake of DNA that can be used as a nutrient source. Similarly, ammonia (NH3) produced by urease activity can be used as a source of nitrogen and, when released from the bacterium, is also toxic for host cells. The activities of Apx toxins include release of heme compounds (iron sources) from red blood cells (RBCs), activation of macrophages and neutrophils which release cytokines (anti‐inflammatory or proinflammatory depending on dose of toxin/stage of infection), killing of these cells, with release of toxic metabolites (including reactive oxygen species that can damage both bacterial and host cells), and direct cytotoxicity for epithelial cells. The poly‐N‐acetyl‐glucosamine (PGA) matrix serves as both an adhesin and as a protective barrier to host immune defenses. For simplicity, a generic nutrient acquisition system including an outer‐membrane transport (OMT) protein and an ATP‐binding cassette (ABC)‐type transport system (AbcABC) is shown in addition to the TonB‐dependent (i.e., requiring energy from TonB‐ExbB‐ExbD) transferrin‐binding receptor (TbpA/TbpB) and the AfuABC proteins, representing one of the iron uptake systems. The trimeric autotransporter adhesins (TAAs) and Flp/Tad pili are not found in all serovars/isolates of A. pleuropneumoniae, but where present, may contribute to adherence. CM, cytoplasmic membrane; LP, lipoprotein; OMP, outer‐membrane protein; PMN, polymorphonuclear leukocytes. The ability of a pathogen to adhere to host cells/surfaces, and to multiply following acquisition of nutrients, is a prerequisite to production of disease (Chapter 1). For A. pleuropneumoniae, pulmonary disease may occur with or without prior tonsil colonization, as inhaled aerosols may directly penetrate the lower respiratory tract. Adherence of A. pleuropneumoniae to epithelial cells and extracellular matrix (ECM) components is likely a complex multifactorial process involving a variety of surface‐exposed proteins and carbohydrates. Adherence assays often employ cultured cell lines, such as St Jude Porcine Lung (SJPL) or A549 human alveolar basal epithelial cells, which although not porcine in origin, appear to be suitable models for detecting differences in adhesion between different A. pleuropneumoniae serovars and/or mutant strains. Among proteinaceous adhesins, surface appendages including a type IV pilus (Tfp), tight‐adherence (Flp/Tad) pilus, and trimeric autotransporter adhesins (TAAs) have been described. Genes encoding Tfp, well conserved in all isolates, are upregulated in vivo and by contact with epithelial cells (Auger et al. 2009; Klitgaard et al. 2012; Deslandes et al. 2010), supporting a role in adherence. The major pilin protein, ApfA, also contributes to host cell adhesion, and recombinant ApfA may improve efficacy of subunit vaccines (Sadílková et al. 2012; Zhou et al. 2013). The genes encoding the Flp/Tad pilus and TAAs are intact only in some serovars/isolates. Although studies with isolates producing these structures have indicated roles in adherence to cultured SJPL cells (T. Li et al. 2012b; Wang et al. 2015a), their relative contributions to virulence differences has not been investigated. The large TAA proteins have ECM‐binding motifs and the Apa1 TAA has been shown to mediate auto‐aggregation, which may facilitate biofilm formation. TAAs contain multiple N‐X‐(S/T) sequons, which are targets of an N‐linked glycosylation system encoded by A. pleuropneumoniae. Deletion of the N‐glycosylation genes reduced adherence of a serovar 15 strain to cultured A549 cells, and it was suggested that N‐glycosylation may stabilize TAA proteins (Cuccui et al. 2017). In addition to protein appendages, surface‐located proteins, including outer‐membrane proteins (OMPs), lipoproteins, and so‐called “moonlighting” proteins (typically cytoplasmic proteins that can also be found on the cell surface) have been implicated in adherence of A. pleuropneumoniae (Chiers et al. 2010; Liu et al. 2018). With availability of genome sequences, the NAD‐restriction‐induced 55 kDa OMP associated with adherence has now been identified as AldA, a cytoplasmic aldehyde dehydrogenase, and its identification might reveal other roles for this protein in adherence. The identity of a putative 60 kDa collagen‐binding OMP is still unknown, as no sequence was determined for the purified protein, and detection of flagella on the bacteria used in the study (Enríquez‐Verdugo et al. 2004) calls into question the results, as no A. pleuropneumoniae isolate has been found to encode flagella. The main surface carbohydrate involved in A. pleuropneumoniae adherence is the biofilm matrix, poly‐N‐acetyl‐glucosamine (PGA), is encoded by the pgaABCD operon (Hathroubi et al. 2018). Expression of PGA involves numerous global regulators including the extracytoplasmic stress sigma factor RpoE, as well as H‐NS, Hfq, (p)ppGpp, and CpxRA. The latter indirectly regulates PGA expression via activation of RpoE, which may also be true for at least some of the OMPs/lipoproteins and membrane components shown to affect biofilm formation. Although best studied in vitro, formation of A. pleuropneumoniae aggregates in the lungs of infected pigs is indicative of biofilm formation in vivo, and initial cultures of most clinical isolates have a biofilm phenotype. Less is known about adhesion in the tonsils where various bacteria co‐exist, and it is not yet known if formation of mixed species biofilms including A. pleuropneumoniae is a factor in tonsil colonization. Clinical lung isolates were recently shown to form biofilms in vitro as frequently as tonsil isolates, with serovar of the isolate more associated with this phenotype than site of isolation (Aper et al. 2020). In contrast to PGA, which is common to all A. pleuropneumoniae, the CPS and O‐Ag surface polysaccharides differ among serovars/isolates. Expression of thick CPS is associated with decreased adherence, likely due to masking of adhesins on the bacterial surface, whereas the role of O‐Ag is less clear. Initially, O‐Ag and not the more conserved LPS core was thought to be the main component mediating A. pleuropneumoniae adherence to frozen porcine tracheal sections, whereas the converse was later shown to be true (Jeannotte et al. 2003). A study of serovar 1 isogenic LPS core and O‐Ag mutants further supported a role for O‐Ag, but not core, in binding to PE, whereas binding to gangliotriaosylceramide (GgO3) and gangliotetraosylceramide (GgO4) was suggested to be mediated by core components, though not via the Gal I‐Hep IV region previously implicated in adhesion to porcine tracheal sections. Recently, one of the serovar 1 O‐Ag mutants showed reduced biofilm formation, with downregulation of cpxRA and pgaA, indicating possible indirect effects of O‐Ag on adhesion (Hathroubi et al. 2018). Following adherence, bacteria need to acquire the necessary nutrients to proliferate and to establish and to maintain colonization/cause disease. Nutrient availability in the lungs is generally low compared with other host niches, necessitating uptake systems for the most limited factors. However, inflammation and tissue injury induced by A. pleuropneumoniae growth and toxin secretion (see below) leads to vascular permeability as well as production of catecholamines, mucus, and neutrophil extracellular traps (NETs), greatly altering the availability of nutrients, as well as generating regions of anoxia and elevated temperature. Mutagenesis and transcriptomic studies indicate the importance of anaerobic respiration in A. pleuropneumoniae, which lacks certain enzymes in the oxidative branch of the tricarboxylic acid cycle. Genes for uptake and metabolism of various carbon sources have been described, with glycerol, ascorbate and maltose suggested to be important for anaerobic growth during lung infection (Klitgaard et al. 2012). Mutation of the transcriptional regulator, malT, induced the stringent response (SR) associated with nutritional starvation, further supporting a key role for maltose/maltodextrins (Lone et al. 2009). Genes upregulated in the malT mutant include those involved in DNA transformation. Extracellular DNA can serve as a source of nutrients (carbon, phosphate, nitrogen, NAD, and purine nucleotides) as well as transforming genetic material. Although only a few naturally transformable isolates have been described, all A. pleuropneumoniae isolates possess the genes required for DNA uptake, and it was recently shown that the presence of NETs (consisting of DNA fibers decorated with antimicrobial proteins), when degraded by host nucleases, enhance growth of A. pleuropneumoniae (de Buhr et al. 2019). Sialic acid (abundant on host mucosal surfaces) may be another important carbon source during acute infection. The coregulated metabolic operon (neuA to nagA) and homologs of the Haemophilus ducreyi sialic acid ABC transport system are reported to be highly expressed early in lung infection (Klitgaard et al. 2012). Konze et al. (2019) have also reported that A. pleuropneumoniae can heterotrophically fix carbon (with the enzymes encoded by pepC and oadA), thus CO2/bicarbonate, abundant in the lung, may represent another important nutrient source. The availability of both branched‐chain amino acids (BCAAs; isoleucine, leucine, and valine) and aromatic amino acids (tryptophan tyrosine and phenylalanine) is low in the host and genes required for their synthesis from precursors are important for viability of A. pleuropneumoniae. All A. pleuropneumoniae genomes encode a putative tryptophan/aromatic amino acid permease gene (tnaB; WP_005612942.1) although the function/importance of this gene has not been explored. Recently, de novo synthesis of vitamin B6 by pyridoxal phosphate synthases PdxS/PdxT was shown to be important for A. pleuropneumoniae survival in a mouse model of infection, suggesting this may also be a limiting nutrient in vivo (Xie et al. 2017a). Trace metals are important cofactors for various metabolic enzymes, but high levels can be toxic so uptake and/or efflux systems are required by bacteria to maintain homeostasis. In the host, free iron concentrations are very low, with most tightly bound by molecules such as transferrin, lactoferrin, haptoglobin, and hemoglobin. The hemolytic activity of Apx toxins (see below) increases the availability of heme compounds (free heme, hemin, hematin, and hemoglobin). A. pleuropneumoniae has acquisition systems for these molecules, as well as for porcine transferrin and siderophores, but not lactoferrin. High‐affinity binding proteins on the outer membrane, with/without associated surface lipoproteins, capture the iron sources, with energy supplied by TonB2‐ExbB2‐ExbD2 (the TonB1‐ExbB1‐ExbD1 system appears less essential) driving uptake into the periplasm. Transport of iron into the cytoplasm is via ABC transport systems (FhuBCD, two AfuABC systems, and a system encoded by WP_039709673/WP_039709399/WP_009875143). The importance of iron acquisition from porcine transferrin (suggested to contribute to host specificity) is demonstrated by attenuation of mutants lacking one or both of the tbpAB genes. Furthermore, catecholamine‐enhanced growth of A. pleuropneumoniae was shown to be mediated via iron acquisition from transferrin (Li et al. 2015). High‐affinity ABC transport systems for uptake of nickel (CbiKLMQO) and zinc (ZnuABC) contribute to establishment of A. pleuropneumoniae infection. Nickel is a cofactor for urease (which produces ammonia, a preferred nitrogen source for many bacteria) and possibly other enzymes. A. pleuropneumoniae genomes encode a number of predicted zinc metalloproteases including the conserved 45 kDa EnvC OMP (WP_005601129), which is a predicted murein hydrolase. There have also been reports of secreted proteases, including a 200 kDa protein (suggested to be a multimer of a 45–47 kDa protein) capable of degrading porcine gelatin and immunoglobulins (Ig), but the gene(s) responsible have not been identified. Both non‐specific and specific components of the respiratory immune system must be overcome to establish infection. There is no direct evidence that A. pleuropneumoniae impairs mucociliary activity, but cytotoxicity mediated by Apx toxins and ammonia (produced by urease activity) could damage ciliated epithelial cells. Bacteria not cleared by normal mucociliary function must avoid elimination by phagocytes (initially macrophages, with increasing numbers of PMNs as the infection progresses). At sub‐lytic doses, the Apx toxins reduce chemotactic and phagocytic activities of macrophages while promoting oxidative metabolism in both macrophages and PMNs. Surface polysaccharides (CPS, O‐Ag, and likely PGA) contribute to antiphagocytic effects and protect cells from complement‐mediated lysis via deposition of complement away from the outer membrane. Following phagocytosis, A. pleuropneumoniae can survive over 90 minutes in macrophages, during which time generation of Apx toxins can lead to apoptosis and the subsequent release of both bacteria and host lytic metabolites that can further lead to tissue damage (see below). Contributing to A. pleuropneumoniae survival in phagocytes, CPS and O‐Ag can scavenge free toxic oxygen radicals while intracellular polyamines (maintained by the PotABCD transport system) may be important for removing reactive oxygen radicals (Zhu et al. 2017). In addition, two superoxide dismutases (SodA and SodC), catalase, and two TolC‐dependent efflux systems (see below) can also detoxify or eliminate toxic oxygen radicals. Urease‐generated ammonia may impair phagosome–lysosome fusion and buffer acid hydrolase activity, and stress–response proteins (discussed below) can mitigate damage. Although transcriptional profiling of A. pleuropneumoniae during macrophage infection indicates upregulation of genes for production of ApxI, LPS and CPS, the genes encoding urease, catalase, and certain stress‐response and DNA repair proteins (LonA, ClpB, HtpX, RadA) are, surprisingly, downregulated (Wang et al. 2015b). Cationic antimicrobial peptides (cathelicidins), particularly abundant in pigs, are important in innate immunity. Studies with the porcine cathelicidin, PR‐39, indicate a putative peptide transport system (encoded by the sap operon), upregulated in the presence of this peptide and in bronchoalveolar fluid, is important for A. pleuropneumoniae resistance, as a sapA mutant showed increased sensitivity to PR‐39 in vitro and was attenuated for virulence in mice (Xie et al. 2017b
12
Actinobacillus
Introduction
Actinobacilluspleuropneumoniae
Source of Infection: Ecology and Epidemiology
Animal host
Actinobacillus spp. or related taxaa
Diseaseb
Horses
A. arthritidis
Septicemia, arthritis
A. equuli subsp. equuli
Septicemia in foals (sleepy foal disease), respiratory infections, abortion, metritis, mastitis, arthritis, pericarditis, meningitis, cellulitis, peritonitis
A. equuli subsp. haemolyticus
Respiratory infections, septicemia, abortion, metritis, mastitis, arthritis, pericarditis, meningitis, cellulitis, peritonitis
A. genomospecies 1c
Stomatitis
A. genomospecies 2 d
Septicemia
Pigs
A. pleuropneumoniae
Fibrinohemorrhagic pneumonia, pleurisy
A. suis
Septicemia, pneumonia, arthritis, enteritis
A. equuli subsp. equuli
Septicemia, abortion, polyarthritis
“A. porcitonsillarum”
Commensal
[A.] indolicus
Commensal, possible opportunist
[A.] minor
Commensal, possible opportunist
[A.] porcinus
Commensal, possible opportunist
[A.] rosii
Abortion, metritis
Ruminants
A. lignieresii
Pyogranulomatous lesions (“wooden tongue”)
Cattle
[A.] succinogenes
Commensal
Sheep
[A.] seminis
Epididymitis, orchitis
Ducks
A. anseriformium
Sinusitis, conjunctivitis, septicemia
Alpaca
A. vicugnae
Septicemia
Rabbits, hares
A. capsulatus
Septicemia, arthritis
Guinea pigs
Bisgaard taxon 8
Commensal
Rodents
[A.] muris
Commensal
Cetacea
[A.] scotia
Commensal
[A.] delphinicola
Commensal
Serovar (K‐Ag)
Biovar
cps typea
O‐Ag/groupb
Toxinsc
Virulence
Country/region
1
1
II
1/9/11
ApxI
ApxII
High
Widespread
2
1
I
2
ApxII
ApxIII
Moderate – high
Widespread
2
2
I
2
ApxII
Low – moderate
Europe
3
1
I
3/6/8/15/17/19
ApxII
ApxIII
Low – moderate
China, Europe
4
1
II
4/7/13/18
ApxII
ApxIII
Low – moderate
Europe, Chile
4
2
II
4/7/13/18
ApxII
Low – moderate
Europe
5a/5b
1
III
5/16
ApxI
ApxII
High
Widespread
6
1
I
3/6/8/15/17/19
ApxII
ApxIII
Low – moderate
Widespread
7
1
I
4/7/13/18
ApxII
Low – moderate
Widespread
7
2
I
4/7/13/18
ApxII
Low – moderate
Europe
8
1
I
3/6/8/15/17/19
ApxII
ApxIII
Low – moderate
Widespread
9
1
I
1/9/11
ApxI
ApxII
High
Europe
9
2
I
1/9/11
ApxI
ApxII
High
Europe
10
1
III
10
ApxI
Low
Europe
11
1
I
1/9/11
ApxI
ApxII
High
Europe
11
2
I
1/9/11
ApxII
moderate
Europe
12
1
II
12
ApxII
Low – moderate
Europe, N. America
13
1
I
10
ApxI
Low – moderate
N. America
13
2
I
13
ApxII
Low – moderate
Europe
14
2
II
14
ApxI
Low
Europe
15
1
II
3/6/8/15/17/19
ApxII
ApxIII
Low – moderate
Widespread
16
1
IV
5/16
ApxI
ApxII
High
Europe
17
1
I
3/6/8/15/17/19
ApxII
Low – moderate
Europe
17
2
I
3/6/8/15/17/19
ApxII
Low – moderate
N. America
18
1
II
4/7/13/18
ApxII
Low – moderate
Europe
19
1
II
3/6/8/15/17/19
ApxII
Low – moderate
Europe
19
1
II
4/7/13/18
ApxII
Low – moderate
N. America
Types of Disease and Pathologic Changes
Pathogenesis
Colonization
Evasion of Host Clearance Mechanisms
Stay updated, free articles. Join our Telegram channel
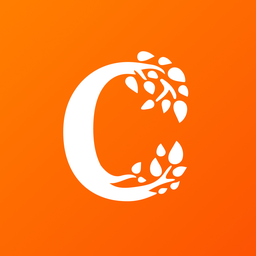
Full access? Get Clinical Tree
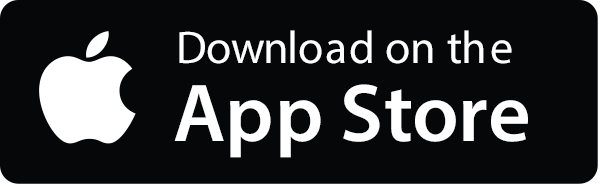
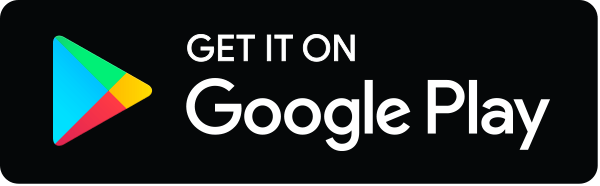