2 Jim E. Riviere The four key physiological processes that govern the time course of drug fate in the body are absorption, distribution, metabolism, and elimination, the so-called ADME processes. Pharmacokinetics, the study of the time course of drug concentrations in the body, provides a means of quantitating ADME parameters. When applied to a clinical situation, pharmacokinetics provides the practitioner with a useful tool to design optimally beneficial drug dosage schedules for each individual patient. In the research and premarketing phase of drug development, it is an essential component in establishing effective yet safe dosage forms and regimens. An understanding of pharmacokinetic principles allows more rational therapeutic decisions to be made. In food animals, pharmacokinetics provides the conceptual underpinnings for understanding and utilizing the withdrawal time to prevent violative drug residues from persisting in the edible tissues of food-producing animals. A working knowledge of this discipline provides the framework upon which many aspects of pharmacology can be integrated into a rational plan for drug usage. To fully appreciate the ADME processes governing the fate of drugs in animals, the various steps involved must be defined and ultimately quantitated. The processes relevant to a discussion of the absorption and disposition of a drug administered by the intravenous (IV), intramuscular (IM), subcutaneous (SC), oral (PO), or topical (TOP) routes are illustrated in Figure 2.1. The normal reference point for pharmacokinetic discussion and analysis is the concentration of free, non-protein-bound drug dissolved in the serum (or plasma), because this is the body fluid that carries the drug throughout the body and from which samples for drug analysis can be readily and repeatedly collected. For the majority of drugs studied, concentrations in the systemic circulation are in equilibrium with the extracellular fluid of well-perfused tissues; thus, serum or plasma drug concentrations generally reflect extracellular fluid drug concentrations. Figure 2.1 Basic schema by which drug is absorbed, distributed, metabolized, and excreted from the body. These processes are those that form the basis for developing pharmacokinetic models. A fundamental axiom of using pharmacokinetics to predict drug effect is that the drug must be present at its site of action in a tissue at a sufficient concentration for a specific period of time to produce a pharmacological effect. Since tissue concentrations of drugs are reflected by extracellular fluid and thus serum drug concentrations, a pharmacokinetic analysis of the disposition of drug in the scheme outlined in Figure 2.1 is useful to assess the activity of a drug in the in vivo setting. This conceptualization is especially important in veterinary medicine where species differences in any of the ADME processes may significantly affect the extent and/or time course of drug absorption and disposition in the body. By dividing the overall process of drug fate into specific phases, this relatively complex situation can be more easily handled. It is the purpose of this chapter to overview the physiological basis of absorption, distribution, metabolism, (biotransformation) and elimination. This will provide a basis for the chapter on pharmacokinetics that will deal with quantitating these processes in more detail. Despite the myriad of anatomical and physiological differences among animals, the biology of drug absorption and distribution, and in some cases even elimination, is very similar in that it involves drug molecules crossing a series of biological membranes. As illustrated in Figure 2.2, these membranes may be associated with either several layers of cells (tissue) or a single cell, and both living and dead protoplasm may be involved. Despite the different biochemical and morphological attributes of each of these membranes, a unifying concept of biology is the basic similarity of all membranes, whether they be tissue, cell, or organelle. Although the specific biochemical components may vary, the fundamental organization is the same. This fact simplifies the understanding of the major determinants of drug absorption, distribution, and excretion. Figure 2.2 Illustration of how absorption, distribution, and excretion is essentially a journey of the drug through various lipoidal membrane barriers. These membrane barriers often directly or indirectly define the nature of compartments or other mathematical modules in pharmacokinetic models. Biological spaces are defined by the restrictions on drug movement imposed by these barriers. The most effective barriers are those that protect the organism from the external environment. These include the skin as well as various segments of the gastrointestinal and respiratory tract, which also protect the internal physiological milieu from the damaging external environment. However, the gastrointestinal and respiratory barriers are modified deep within the body to allow for nutrient and gas exchange vital for life. The interstitial fluid is a common compartment through which any drug must transit, either after absorption on route to the blood stream or after delivery by blood to a tissue on route to a cellular target. Capillary membranes interfacing with this interstitial fluid compartment are relatively porous due to the fenestrae that allow large molecules to exchange between tissues and blood. Membranes define homogeneous tissue compartments and membranes must be traversed in all processes of drug absorption and disposition. All cellular membranes appear to be primarily lipid bilayers into which are embedded proteins that may reside on either surface (intra- or extracellular) or traverse the entire structure. The lipid leaflets are arranged with hydrophilic (polar) head groups on the surface and hydrophobic (nonpolar) tails forming the interior. The specific lipid composition varies widely across different tissues and levels of biological organization. The location of the proteins in the lipid matrix is primarily a consequence of their hydrophobic regions residing in the lipid interior and their hydrophilic and ionic regions occupying the surface. This is thermodynamically the most stable configuration. Changes in the fluidity of the lipids alter protein conformations, which then may modulate their activity. This was one of the mechanisms of action proposed for gaseous anesthetics, although recently specific protein receptors have now been suggested. In some cases, aqueous channels form from integral proteins that traverse the membrane. In other cases, these integral proteins may actually be enzymatic transport proteins that function as active or facilitative transport systems. The primary pathway for drugs to cross these lipid membranes is by passive diffusion through the lipid environment. Thus, in order for a drug to be absorbed or distributed throughout the body, it must be able to pass through a lipid membrane on some part of its sojourn through the body. In some absorption sites and in many capillaries, fenestrated pores exist, which allow some flow of small molecules. This is contrasted to some protected sites of the body (e.g., brain, cerebral spinal fluid) where additional membranes (e.g., glial cells) may have to be traversed before a drug arrives at its target site. These specialized membranes could be considered a general adaptation to further shelter susceptible tissues from hostile lipophilic chemicals. In this case, drug characteristics that promote transmembrane diffusion would favor drug action and effect (again unless specific transport systems intervene). This general phenomenon of the enhanced absorption and distribution of lipophilic compounds is a unifying tenet that runs throughout the study of drug fate. The body’s elimination organs can also be viewed as operating along a somewhat similar principle. The primary mechanism by which a chemical can be excreted from the body is by becoming less lipophilic and more hydrophilic, the latter property being required for excretion in the aqueous fluids of the urinary or biliary systems. When a hydrophilic or polar drug is injected into the bloodstream, it will be minimally distributed and rapidly excreted by one of these routes. However, if a compound’s lipophilicity evades this easy excretion, the liver and other organs may metabolize it to less lipophilic and more hydrophilic metabolites that have a restricted distribution (and thus reduced access to sites for activity) in the body and can be more readily excreted. This basic tenet runs throughout all aspects of pharmacology and is a useful concept to predict effects of unknown compounds. Considerable evidence exists that lipid-based membranes are permeable to nonpolar lipid-soluble compounds and polar water-soluble compounds with sufficient lipid solubility to diffuse through the hydrophobic regions of the membrane. The rate of diffusion of a compound across a membrane is directly proportional to its concentration gradient across the membrane, lipid\water partition coefficient, and diffusion coefficient. This can be summarized by Fick’s law of diffusion in Equation 2.1: where D is the diffusion coefficient for the specific penetrant in the membrane being studied, P is the partition coefficient for the penetrant between the membrane and the external medium, h is the thickness or actual length of the path by which the drug diffuses through the membrane, and X1 − X2 is the concentration gradient (ΔX) across the membrane. The diffusional coefficient of the drug is a function of its molecular size, molecular conformation and solubility in the membrane milieu, and degree of ionization. The partition coefficient is the relative solubility of the compound in lipid and water that reflects the ability of the penetrant to gain access to the lipid membrane. Depending on the membrane, there is a functional molecular size and/or weight cutoff that prevents very large molecules from being passively absorbed across any membrane. When the rate of a process is dependent upon a rate constant (in this case [DP/h] often referred to as the permeability coefficient P) and a concentration gradient, a linear or first-order kinetic process is evident (see Chapter 3 for full discussion). In membrane transfer studies, the total flux of drug across a membrane is dependent on the area of membrane exposed; thus the rate above is often expressed in terms of cm2. If the lipid : water partition coefficient is too great, depending on the specific membrane, the compound may be sequestered in the membrane rather than traverse it. Evidence also exists that membranes are more permeable to the nonionized than the ionized form of weak organic acids and bases. If the nonionized moiety has a lipid : water partition coefficient favorable for membrane penetration, it will ultimately reach equilibrium on both sides of the membrane. The ionized form of the drug is completely prevented from crossing the membrane because of its low lipid solubility. The amount of the drug in the ionized or nonionized form depends upon the pKa (negative logarithm of the acidic dissociation constant) of the drug and the pH of the medium on either side of the membrane (e.g., intracellular versus extracellular fluid; gastrointestinal versus extracellular fluid). Protonated weak acids are nonionized (e.g., COOH) while protonated weak bases are ionized (e.g., NH3+). If the drug has a fixed charge at all pHs encountered inside and outside of the body (e.g., quarternary amines, aminoglycoside antibiotics), they will never cross lipid membranes by diffusion. This would restrict both their absorption and distribution and generally lead to an enhanced rate of elimination. It is the nonionized form of the drug that is governed by Fick’s Law of Diffusion and described by Equation 2.1 above. For this equation to predict the movement of a drug across membrane systems in vivo, the relevant pH of each compartment must be considered relative to the compound’s pKa; otherwise, erroneous predictions will be made. When the pH of the medium is equal to the pKa of the dissolved drug, 50% of the drug exists in the ionized state and 50% in the nonionized, lipid-soluble state. The ratio of nonionized to ionized drug is given by the Henderson–Hasselbalch equation (Equations 2.2 and 2.3).For acids: For bases: These equations are identical as they involve the ratio of protonated (H) to nonprotonated moieties. The only difference is that for an acid, the protonated form (H Acid)0 is neutral while for a base, the protonated form (H Base)+ is ionized. This topic is also presented in Chapter 5 (Equations 5.1 to 5.5; Figure 5.3). As can be seen by these equations, when the pH is one unit less or one unit more than the pKa for weak bases or acids, respectively, the ratio of ionized to nonionized is 10. Thus each unit of pH away from the pKa results in a tenfold change in this ratio. This phenomenon allows for a drug to be differentially distributed across a membrane in the presence of a pH gradient, an effect that often dwarfs that obtainable by increasing dose to increase drug delivery to a specific tissue. The side of the membrane with the pH favoring ionized drug (high pH for an acidic drug; low pH for an alkaline drug) will tend to have higher total (ionized plus nonionized) drug concentrations. This pH partitioning results in so-called “ion trapping” in the area where the ionized drug predominates. Figure 2.3 illustrates this concept with an organic acid of pKa = 3.4 partitioning between gastric contents of pH = 1.4 and plasma of pH = 7.4. Assuming that the nonionized form of the drug (U) is in equilibrium across the membrane, then, according to Equations 2.1 and 2.2, there will be a 100-fold (log 2; 3.4–1.4) difference on the gastric side and a 10,000-fold (log 4; 7.4–3.4) difference on the plasma side of the membrane, for a transmembrane concentration gradient of total drug (U plus I) equal to 10,001/1.01. Note that the unionized concentration on both sides of the membrane are in equilibrium. It is the total drug concentrations that are different. In this case, the gradient is generated by the difference in pH across an ion-impermeable barrier generated by the local milieu. Figure 2.3 The phenomenon of pH partitioning and ion trapping of a weak acid. Such a gradient would greatly favor the absorption of this weak acid across the gastrointestinal tract into plasma. This is the situation that exists for weak acids such as penicillin, aspirin, and phenylbutazone. In contrast, a weak base would tend to be trapped in this environment and thus minimal absorption would occur. Examples of such weak bases are morphine, phenothiazine, and ketamine. Specific active transport systems may counter these predictions (e.g., β-lactam transporters in intestines), as well as the extreme surface area of the small intestines compared to gastric mucosa, which generally favors absorption of most drugs in the small intestines. With the weakly basic strychnine, pH-dependent absorption is toxicologically significant. If strychnine were placed into the strongly acidic stomach, no systemic toxicity would be observed. However, if the stomach were then infused with alkali, most of this base would become nonionized, readily absorbed, and lethal. In summary, weak acids are readily absorbed from an acid environment and sequestered in an alkaline medium. In contrast, weak bases are absorbed in an alkaline environment and trapped in an acidic environment. This pH partitioning phenomenon is not only important in understanding absorption (as illustrated above), but also in any situation where the pH of fluid compartments across a biological membrane is different. It will occur for a drug distributing from plasma (pH = 7.4) to milk (pH = 6.5–6.8), to cerebrospinal fluid (pH = 7.3), or to intracellular sites (pH = 7.0). Thus weakly acidic drugs will tend not to distribute into the milk after systemic distribution (e.g., penicillin), while weakly basic drugs (e.g., erythromycin) will. If a disease process alters the pH of one compartment (e.g., mastitis), the normal equilibrium ratio will also be perturbed. In mastitis, where pH may increase almost one unit, this preferential distribution of basic antibiotics will be lost. The relatively acidic pH of cells relative to plasma is responsible for the relatively large tissue distribution seen with many weakly basic drugs (e.g., morphine, amphetamine). Similarly, in the ruminant, many basic drugs tend to distribute into the rumen, resulting in distribution volumes much larger than those in monogastrics. In fact, a drug that distributes into this organ may then undergo microbial degradation resulting in its elimination from the body. This phenomenon is also very important for the passive tubular reabsorption of weak acids and bases being excreted by the kidney. For carnivores with acidic urine relative to plasma, weak acids tend to be reabsorbed from the tubules into the plasma while weak bases tend to be preferentially excreted. This principle has been applied to the treatment of salicylate (weak acid) intoxication in dogs where alkaline diuresis promotes ion trapping of the drug in the urine and hence its rapid excretion. Disease-induced changes in urine pH will likewise alter the disposition of drugs sensitive to this phenomenon. Movement across the fenestrated capillaries of the body from plasma to tissue areas generally allows movement of most drugs. In these cases, relatively small molecules (molecular weights <1,000) can pass through independent of their lipid solubility, but larger molecules are excluded. In all of these scenarios, drugs move through these tissues as a solute dissolved in water and essentially are transported wherever the water goes. This process is termed bulk flow and is dependent on the concentration of drug dissolved in the plasma or tissue fluid. This is a linear process and thus is easily modeled by most pharmacokinetic systems. It is the subsequent uptake into cells and special tissue areas that are governed by the diffusion processes above. There exist several specialized membranes that possess specific transport systems. In these cases, the laws of diffusion and pH partitioning do not govern transmembrane flux of drugs. These specializations in transport can best be appreciated as mechanisms by which the body can exert control and selectivity over the chemicals that are allowed to enter the protected domain of specific organs, cells, or organelles. Such transport systems can be rather nonspecific, as are those of the kidney and liver that excrete charged waste products. In the gastrointestinal tract, relatively nonspecific transport systems allow for the absorption, and thus entrance to the body, of essential nutrients that do not have sufficient lipophilicity to cross membranes by diffusion. In specific tissues, they allow for select molecules to enter cells depending upon cellular needs, or allow compounds that circulate throughout the body to have a biological response only in a tissue possessing the correct transport receptor. The primary example is the protein carrier-mediated processes of active transport or facilitated diffusion. These systems are characterized by specificity and saturability. In the case of active transport, biological energy is utilized to move a drug against its concentration gradient. In facilitated diffusion, the carrier protein binds to the drug and carries it across the membrane down its concentration gradient. The drugs transported by these systems normally cannot cross the membrane by passive diffusion because they are not lipophilic. These systems are important for the gastrointestinal absorption of many essential nutrients and some drugs (e.g., β-lactams), for cellular uptake of many compounds (e.g., glucose), for the removal of drugs from the cerebral spinal fluid through the choroid plexus, and for biliary and renal excretion of numerous drugs. In some tissues, cells may absorb drugs by endocytosis or pinocytosis, processes where a compound binds to the surface of the membrane that then invaginates and interiorizes the compound. This is not a primary mechanism of transmembrane passage for most therapeutic drugs. It is the primary mechanism by which nanomaterials enter cells. Most inorganic ions such as sodium and chloride are sufficiently small that they easily can cross aqueous pores and channels through membranes. The movement of these charged substances is generally governed by the transmembrane electrical potential maintained by active ion pumps. Finally, active transport may also occur in the opposite direction to remove a drug after it has been absorbed into specific cells or tissue sites. This is called the P-glycoprotein system, a class of drug transporters originally associated with multiple drug resistance (MDR) encountered in cancer chemotherapy. MDR transporters have been identified in intestinal epithelial cells, the placenta, kidney tubules, brain endothelial cells, and liver bile canaliculus. These will be addressed throughout this text for specific drug classes. In conclusion, an understanding of the processes that govern the movement of drugs across lipid-based biological membranes is important to the study of drug absorption, distribution, and excretion. Lipid-soluble drugs are easily absorbed into the body and well distributed throughout the tissues. In contrast, hydrophilic drugs are not well absorbed and have limited distribution but are easily eliminated. Metabolism converts lipophilic drugs to more easily excreted hydrophilic entities. If membranes separate areas of different pH, concentration gradients may form due to pH partitioning or ion trapping. Membranes are the building blocks of biological systems and play a central role in defining the complexity of pharmacokinetic models. Absorption is the movement of the drug from the site of administration into the blood. There are a number of methods available for administering drugs to animals. The primary routes of drug absorption from environmental exposure in mammals are gastrointestinal, dermal, and respiratory. The first two are also used as routes of drug administration for systemic effects, with additional routes including intravenous, intramuscular, subcutaneous, or intraperitoneal injection. Other variations on gastrointestinal absorption include intrarumenal, sublingual, and rectal drug delivery. Many techniques are also used for localized therapy, which may also result in systemic drug absorption as a side effect. These include, among others: topical, intramammary, intraarticular, subconjunctival, and spinal fluid injections. Methods of utilizing these different routes of drug administration are also explored in Chapter 5. One of the primary routes of drug administration is oral ingestion of a pill or tablet that is designed to deliver a drug across the gastrointestinal mucosa. The common factor in all forms of oral drug administration is a method to deliver a drug such that it gets into solution in the gastrointestinal fluids from which it can then be absorbed across the mucosa and ultimately reach the submucosal capillaries and the systemic circulation. Examples of oral drug delivery systems include solutions (aqueous, elixirs) and suspensions, pills, tablets, boluses for food animals, capsules, pellets, and sustained release mechanical devices for ruminants. The major obstacle encountered in comparative and veterinary medicine is the enormous interspecies diversity in comparative gastrointestinal anatomy and physiology, which results in major species differences in strategies for and efficiency of oral drug administration. This is often appreciated but overlooked when laboratory animal data is extrapolated to humans. Rats and rabbits are widely utilized in preclinical disposition and toxicology studies, although many investigators fail to appreciate that these species’ gastrointestinal tracts are very different from one another and from humans. From a pharmacologist’s perspective, the gastrointestinal tract of all species can be simply presented as diagrammed in Figure 2.4. The GI tract is best conceptualized as actually being part of the external environment, which, in contrast to the skin, is protected and whose microenvironment is closely regulated by the organism. Because of the gastrointestinal tract’s central role in digestion and nutrient absorption, there are many evolutionary adaptations to this basically simple mucosa structure that allow for physical, chemical, enzymatic, and microbial breakdown of food for liberation and ultimate absorption of nutrients. This tract is further adapted such that these digestive processes do not harm the organism’s own tissues, which in carnivores may be identical to the food being eaten. Figure 2.4 Functional structure of the gastrointestinal tract. The gastrointestinal tract presents a significant degree of heterogeneity relative to morphology and physiology that translates to great regional variations in drug absorption. In the oral cavity, where food is masticated, some absorption may occur in sublingual areas. In fact, this site is actually utilized as a route for systemic drug (e.g., nitroglycerin) and nicotine (e.g., oral tobacco) delivery in humans. The esophagus and cranial portion of the stomach is lined by cornified epithelium, which provides an effective barrier that often decreases the chance of absorption for drugs formulated for intestinal drug delivery. A great deal of recent research activity has been focused on developing new transbuccal drug delivery systems. As mentioned, the prototype example was sublingual nitroglycerin tablets. Newer systems use novel adhesive technology, which allow actual polymer patches to adhere to the buccal mucosa. Such products are also being considered for some therapeutic applications in veterinary medicine (e.g., feline oral sprays). Compared to oral gastrointestinal absorption, buccal delivery bypasses the portal vein and thus eliminates the potential for first-pass hepatic biotransformation, discussed later. The simple mucosal lining of the stomach allows absorption; however, the presence of surface mucus, which protects the epithelium from self-digestion secondary to acid and enzyme secretion, may be a barrier for some drugs. The acidity and motility of the stomach also creates a hostile environment for drugs and even influences the absorption of drugs farther down the tract. For oral drug absorption to be successful, the drug must be capable of surviving this relatively harsh environment. For some drugs (e.g., penicillin G) susceptible to acid hydrolysis, minimal absorption by the oral route will occur unless they are administered in a formulation that protects them in an acid environment but liberates them in the more alkaline environment of the intestines. Release of the drug from the stomach, a process controlled by gastric emptying, is a major rate-determining step in the onset and duration of oral drug activity. Species differences in the size of the pyloric orifice also limit use of some dosage forms in small animals versus humans. The primary site for most drug absorption is the small intestine. In this region of the gastrointestinal tract, the pH of the contents are more alkaline and the epithelial lining is conducive to drug absorption. The blood flow to this region is also much greater than to the stomach. The small intestine is lined by simple columnar epithelium resting on a basement membrane and a submucosal tissue bed that is very well perfused by an extensive capillary and lymphatic network. This capillary bed drains into the hepatic portal vein. One of the major anatomical adaptations for absorption in this region is the presence of microvilli, which increase the surface area of the small intestine some 600-fold over that of a simple tube. The second anatomical adaptation are the villi of the intestine, which can be easily appreciated by examining a cross section (Figure 2.5). Since diffusion is the primary mechanism for drug absorption, the increase in area due to these two anatomical configurations significantly increases absorption, as can be seen from reviewing the area contribution to Equation 2.1. There are species differences in inherent permeability of the intestinal mucosa to chemicals, with the dog recently being recognized as having a higher permeability to many drugs than humans. Figure 2.5 Cross-section of the small intestine showing villi adaptations, which serve to increase surface area available for absorption. The viable epithelial cells of the intestines are also endowed with the necessary enzymes for drug metabolism that contributes to a second “first-pass” effect. Recent research has also indicated that the mechanism and extent of absorption, and magnitude of local intestinal metabolism, varies between the tips and crypts of the villi. The final determinant of a drug’s tortuous journey through the gastrointestinal tract is the resident microbial population that inhabits the intestinal contents. Many bacteria are capable of metabolizing specific drugs, resulting in a third component of the first-pass effect. This epithelial and bacterial biotransformation is generally categorized as presystemic metabolism to differentiate it from that which occurs following portal vein delivery of drug to the liver. However, from the perspective of pharmacokinetic analysis of plasma drug concentrations following oral drug administration, all three components are indistinguishable and become lumped into the aggregate process of oral absorption assessed as Ka. In order for a drug to be absorbed across the intestinal mucosa, the drug must first be dissolved in the aqueous intestinal fluid. Two steps, disintegration and dissolution, may be required for this to occur. Disintegration is the process whereby a solid dosage form (e.g., tablet) physically disperses so that its constituent particles can be exposed to the gastrointestinal fluid. Dissolution occurs when the drug molecules then enter into solution. This component of the process is technically termed the pharmaceutical phase and is controlled by the interaction of the formulation with the intestinal contents. These concepts are further elaborated on in Chapter 5. Some dosage forms, such as capsules and lozenges, may not be designed to disintegrate, but rather to allow a drug to slowly elute from their surface. Dissolution is often the rate-limiting step controlling the absorption process and can be enhanced by formulating the drug in salt form (e.g., sodium or hydrochloride salts), buffering the preparation (e.g., buffered aspirin), or decreasing dispersed particle size (micronization) so as to maximize exposed surface area. This is extensively discussed in Chapter 5 (see equation 5.7). Alternatively, disintegration and dissolution can be decreased so as to deliberately provide slow release of the drug. This strategy is used in prolonged-release or controlled-release dosage forms and involves complex pharmaceutical formulations that produce different rates of dissolution. This may be accomplished by dispersing the dosage form into particles with different rates of dissolution or by using multilaminated dosage forms, which delays release of the drug until its layer is exposed. All of these strategies decrease the overall rate of absorption. Similar strategies can also be used to target drugs to the distal segments of the gastrointestinal tract by using enteric coatings that dissolve only at specific pH ranges, thereby preventing dissolution until the drug is in the region targeted. This strategy has been applied for colonic delivery of drugs in humans for treatment of Crohn’s disease. In slow-release or long-acting formulations, the end result is that absorption becomes slower than all other distribution and elimination processes, making the pharmaceutical phase the rate-limiting or rate-controlling step in the subsequent absorption and disposition of the drug. When this occurs, as will be seen in the pharmacokinetic modeling chapters to follow, the rate of absorption controls the rate of apparent drug elimination from the body and a so-called flip-flop scenario becomes operative. There are significant species differences in the ability to use controlled-release oral medications designed in humans, by far the largest market, in other species. The first limitation involves the inability to use cellulose-based systems in ruminants due to the ability of rumen microbes to digest the normally inert cellulose matrix that controls rates of drug delivery. The second arises because of shorter gastrointestinal transit times in small carnivores, such as domestic cats and dogs, compared to humans. In this instance, drug release is designed to occur in the longer transit times seen in humans (approx. 24 hours). In dogs and cats, which have transit times half that of humans, drug release may still be occurring even after the tablet has been eliminated in the feces due to the shorter transit times. Other examples include the narrower pyloric opening in dogs, compared to humans, that may increase gastric retention of some larger dosage forms. These are but a few examples of significant species differences that, based on anatomical and physiological factors, prevent the ready transferability of complex dosage forms across species. After the drug is in solution, it must still be in a nonionized relatively lipid-soluble form to be absorbed across the lipid membranes comprising the intestinal mucosa. It must be stressed that absorption across any membrane is a fine balance between adequate solubility on the donor side of the membrane with sufficient permeability (or active transport capacity) to actually transit the membrane. For orally administered products, the pH of the gastrointestinal contents becomes very important, as is evident from the earlier discussion on pH partitioning. Specifically, a weak acid would tend to be preferentially absorbed in the more acidic environment of the stomach since a larger fraction would be in the nonionized form. However, the much larger surface area and blood flow available for absorption in the more alkaline intestine may override this effect. It is important to mention at this point why a weak acid such as aspirin is better absorbed in a bicarbonate buffered form, which would tend to increase the ionized fraction and thus decrease membrane passage. The paradox is that dissolution must first occur, a process favored by the ionized form of the drug. It is only the dissolved ionized aspirin that is available to the partitioning phenomenon described earlier. Thus, when more aspirin is dissolved in the buffered microenvironment, more is available for partitioning and diffusion across the mucosa. In contrast to the situation of a weak acid, a weak base would tend to be better absorbed in the more alkaline environment. However, it must be repeated that the very large surface area available in the intestines, coupled with high blood flow and a pH of approximately 5.3 in the immediate area of the mucosal surface makes it the primary site of absorption for most drugs (weak acids with pKa >3 and weak bases with pKa <7.8). Species differences in both gastric and intestinal pH further modulate this differential (e.g., canine gastric pH is much higher than humans). A further obstacle to absorption is that the compound must also be structurally stable against chemical or enzymatic attack. Finally, compounds with a fixed charge and/or very low (or very high) lipid solubility for the uncharged moiety, may not be significantly absorbed after oral administration. Examples include the polar aminoglycoside antibiotics, the so-called “enteric” sulfonamides, and quaternary ammonium drugs. There are also specific active transport systems present within the intestinal mucosa of the microvilli that are responsible for nutrient absorption. However, these systems have a very high capacity and if a specific drug or toxicant has the proper molecular configuration to be transported, saturation is unlikely. There is some evidence that select therapeutic drugs (e.g., β-lactams such as ampicillin) may be absorbed by active transport systems in the small intestine. There are also transport systems (P-glycoprotein) that expel absorbed drug back into the intestinal lumen. This system is beginning to be studied more closely in veterinary species and will be discussed later in this chapter under distribution and elimination. The complexity of these processes is clearly seen when one tries to extrapolate oral bioavailability of drugs between dogs and humans. In order to try and classify drug absorption in humans based on criteria of solubility and permeability, the Biopharmaceutical Classification System (BCS) was developed, which ranked drugs according to their extent and rate of absorption, with Class I drugs being both highly soluble and permeable resulting in generally very well absorbed compounds, in contrast to Class IV which had low solubility and low permeability and showed very poor oral absorption. Class II and III have mixed solubility and permeability characteristics. The advantage of such classifications is that manufactures changing formulations for Class I drugs only need to conduct in vitro dissolution studies since dissolution would be the rate-limiting process in absorption. Class IV drugs would require in vivo comparisons. Drugs classified using this system for humans did not correlate to what was observed in dogs, suggesting that the differences in GI physiology discussed above prevents easy interspecies extrapolations (Papich and Martinez, 2015). The gastrointestinal tract has also evolved into an excretory organ for elimination of nonabsorbed solid wastes and other metabolic byproducts excreted in the bile. The bile duct drains into the upper small intestine. For some drugs, this results in a phenomenon called enterohepatic recycling whereby a drug from the systemic circulation is excreted into the bile and is reabsorbed from the small intestine back into the bloodstream. In many cases, drugs that are metabolized by Phase II conjugation reactions are “unconjugated” by resident bacterial flora, which generates free drug for reabsorption. Thus compounds that are excreted into the bile may have a prolonged sojourn in the body because of the continuous opportunity for intestinal reabsorption. The cardinal sign of this process is a “hump” in the plasma drug concentration-time profile after administration (Figure 2.6). Bile also serves to emulsify fatty substances that are not capable of solubilizing in the primarily aqueous environment of the intestines. The result of this detergent-like action of bile is to form large surface area micelles having a hydrophilic surface and hydrophobic interior. These act as transport vehicles to deliver fat-soluble drugs to the intestinal brush border surface for diffusion across the lipid membrane into the cell. Without the interaction of bile acids, fatty substances would not be available for absorption since they could not traverse this “dissolution” barrier. Thus unlike most drugs, compounds that are absorbed by this route often must be administered with a meal to promote bile acid secretion and associated micelle formation. Figure 2.6 Concentration versus time profile demonstrating a secondary peak that could result from enterohepatic recycling. Enterohepatic recycling has also been suggested as an important mechanism for enhanced activity of certain antiparasitic drugs such as the avermectins. As discussed in Chapter 41, the recycling of active drug is a major contributor to enhanced parasite exposure. Finally, some species such as rabbits, routinely ingest fresh feces for nutritional purposes, which provides another opportunity for drug to be reabsorbed into the body. Food may also interact with other aspects of oral drug absorption and have opposite effects for more hydrophilic drugs. Depending on the physicochemical properties of the specific drug, administration with food may significantly increase or decrease absorption. Such effects are not only drug dependent, but also are species dependent due to the continuous foraging behavior of ruminants and some other omnivores compared to the periodic feeding habits of predatory carnivores. These variables are difficult to incorporate into formal pharmacokinetic models yet they add to the variability in parameters derived from these studies or in drug response between species. The first potential interaction relates to the rate of drug delivery to the small intestine that is governed by the rate of drug release from the stomach, the gastric emptying time. This process is dependent upon the eating habits of the species, with continuous foraging animals (e.g., herbivores such as horses and ruminants) having a steady input of drug and a relatively stable gastric pH compared to periodic eaters (e.g., carnivores like dogs and cats and omnivores such as pigs) who have more variable eating patterns with large swings in gastric pH depending on the presence or absence of food. In addition, the drug may directly interact with the ingested food, as is the case of chelation of tetracyclines with divalent cations such as Mg++ in antacids or Ca++ in milk products. Thus, the decision to administer a compound with or without food is species and drug dependent and may significantly alter the bioavailability (rate and extent of absorption) of the drug. In contrast, for very-lipid-soluble drugs, food is necessary in order to have bile release, which allows solubilization and absorption to occur. The forestomachs of a ruminant provide a major obstacle to the delivery of an oral dosage form to the true stomach (abomasum) for ultimate release to the intestines, although a significant amount of drug absorption may occur from this site. The rumen is essentially a large fermentation vat (>50 liters in cattle, 5 liters in sheep) lined by stratified squamous epithelium, buffered at approximately a pH of 6 by extensive input of saliva, which maintains it in a fluid to soft consistency, designed primarily for the absorption of volatile fatty acids. If drugs dissolve in this medium and remain intact, they undergo tremendous dilution that decreases their rate of absorption. They then are pumped from the rumen and reticulum through the omasum for a rather steady input of drug into the true stomach. An understanding of the physiology of the ruminant has allowed for the development of some unique and innovative mechanical drug delivery technologies, which essentially are encapsulated pumps that “sink” to the bottom of the rumen and become trapped, much as many unwanted objects tend to when ingested by a ruminant (e.g., nails and wire in hardware disease). These “submarine-like” devices then slowly release drug into the ruminal fluid for a true sustained-release preparation. In preruminant calves, a drug may bypass the rumen entirely through the rumen-reticulo groove and essentially behave as if administered to a monogastric. In contrast, fermentation in the horse occurs after drug absorption by the small intestine and thus has less impact than in ruminants. However, a nonabsorbed drug that reaches the equine large intestines and cecum, the site of fermentation, may have disastrous effects (e.g., colic) if digestive flora or function is perturbed. Another unique aspect of oral drug absorption is the fate of the absorbed drug once it enters the submucosal capillaries. Drug absorbed distal to the oral cavity and proximal to the rectum in most species enters the portal circulation and is transported directly to the liver where biotransformation may occur. This is a major cause for differences in a drug’s ultimate disposition compared to all other routes of administration. This may result in a significant first-pass biotransformation of the absorbed compound. For a drug that is extensively metabolized by the liver, this first-pass effect significantly reduces absorption of the active drug even when it is absorbed across the mucosa. This occurs for many opiate medications in dogs, reducing their efficacy after oral administration. Finally, some drugs that are too polar to be absorbed across the gastrointestinal wall are formulated as ester conjugates to increase lipid solubility and enhance absorption. Once the drug crosses the gastrointestinal epithelium in this form, subsequent first-pass hepatic biotransformation enzymes and circulating blood and mucosal esterases cleave off the ester moiety releasing free drug into the systemic circulation. There are selected drug administration sites that avoid first-pass hepatic metabolism by allowing absorption through gastrointestinal tract segments not drained by the portal vein. These include the oral cavity buccal and rectal routes of drug administration in some species, although this assumption hasn’t been tested in many veterinary species. The pharmaceutical literature is replete with formulation factors that may influence the dissolution and absorption of a drug preparation, assuming in the first place that one has an active component of known purity and potency. These are fully discussed in Chapter 5. The issue then becomes what are the potential interactions that can occur between the active ingredients and the excipients that make up the formulation. Additionally, what are the effects of the practitioner’s compounding techniques (materials used, mixing efficacy, etc.) on the amount of active ingredients ultimately appearing in the formulation. Although this discussion is the focus of a biopharmaceutics text, the strategies are often encountered in pharmacokinetics as they may affect the parameters estimated after oral administration. Table 2.1 depicts the pharmaceutical processes involved in absorption that may be affected by formulation. Following oral administration of tablets, disintegration must first occur. The speed and efficacy of this process will determine how much drug is actually available for subsequent steps. The resulting particle size (and hence surface area) is an important determinant for the next dissolution phase where the drug enters solution, an absolute prerequisite for diffusion across the mucosal barrier. Dissolution also involves diffusion across the liquid boundary layers that are an interface between the particles and the absorption milieu. Many pharmaceutical factors may affect the efficiency of the disintegration and dissolution processes. For tablets, the nature and homogeneity of the excipients become important considerations. These factors are the primary determinants of differences in efficacy between so-called “pioneer” and generic drug products. Once the drug is in solution, binding or complexation to inert filler ingredients may occur. It is important to remember that all of this is happening while the particles are in transit through the gastrointestinal tract. Thus, if the formulation results in a decreased rate of disintegration or dissolution, the rate and extent of absorption may be decreased, especially in species with very short gastrointestinal transit times. Similar factors are involved with oral capsules and even liquid dosage forms where the drug may interact with the vehicle. In fact, these scenarios are probably most pertinent to practitioner compounding. For capsules, the breakdown of the capsule replaces tablet disintegration as the initial rate-determining step. After release of the capsule contents, all of the above factors come into play. It cannot be overstated that such pharmaceutical factors are critical determinants of the extent and rate of subsequent drug absorption. Table 2.1 Pharmaceutical factors affecting absorption The skin is a complex, multilayered tissue comprising 18,000 cm2 of surface in an average human male. The quantitative prediction of the rate and extent of percutaneous penetration (into skin) and absorption (through skin) of topically applied chemicals is complicated by the biological variability inherent in skin. Mammalian skin is a dynamic organ with a myriad of biological functions. The most obvious is its barrier property that is of primary concern in the absorption problem. Another major function is thermoregulation that is achieved and regulated by three mechanisms in skin: thermal insulation provided by pelage and hair, sweating, and alteration of cutaneous blood flow. Other functions of skin include mechanical support, neurosensory reception, endocrinology, immunology, and glandular secretion. These additional biological roles lead to functional and structural adaptations that affect the skin’s barrier properties and thus the rate and extent of percutaneous absorption. Many of the topics discussed below are fully developed in Chapter 47 of this text. The skin is generally considered to be an efficient barrier preventing absorption (and thus systemic exposure) of most topically administered compounds. It is a membrane that is relatively impermeable to aqueous solutions and most ions. It is, however, permeable in varying degrees to a large number of solid, liquid, and gaseous xenobiotics. Although one tends to think of most cases of poisoning as occurring through the oral or, less frequently, the respiratory route, the widespread use of organic chemicals has enhanced risk exposure to many toxicants that can penetrate the dermal barrier. The gross features of mammalian skin are illustrated in Figure 2.7. Compared to most routes of drug absorption, the skin is by far the most diverse across species (e.g., sheep versus pig) and body sites (e.g., human forearm versus scalp). Three distinct layers and a number of associated appendages make up this nonhomogenous organ. The epidermis is a multilayered tissue varying in thickness in humans from 0.15 mm (eyelids) to 0.8 mm (palms). The primary cell type found in the epidermis is the keratinocyte. Proliferative layers of the basal keratinocyte (stratum germinativum) differentiate and gradually replace the surface cells (stratum corneum) as they deteriorate and are sloughed from the epidermis. A number of other cell types are also found interspersed in the epidermis including the pigmented melanocytes, Merkel cells which may play a sensory role, and Langerhans cells which probably play a role in cutaneous immunology. Figure 2.7 Microstructure of mammalian skin showing potential routes of penetration (A) intercellular, (B) transcellular, (C) intrafollicular, (D) via sweat ducts. In respect to drug penetration, the primary biochemical change is the production of fibrous, insoluble keratin that fills the cells, and a sulfur-rich amorphous protein that comprises the cell matrix and thickened cell membrane. In addition, the keratinocytes synthesize a variety of lipids that form the distinguishing granules in the stratum granulosum that release their contents into the intercellular spaces. The end result in the stratum corneum is dead proteinaceous keratinocytes embedded in an extracellular lipid matrix, a structure referred to by Elias as the “brick and mortar” model (see Figures 47.3 and 47.4). It is this outermost layer, the stratum corneum, which provides the primary barrier to the penetration of foreign compounds. This barrier consists of flattened, stratified, highly keratinized cells embedded in a lipid matrix composed primarily of sterols, other neutral lipids, and ceramides. Although highly water retarding, the dead, keratinized cells are highly water absorbent (hydrophilic), a property that keeps the skin supple and soft. A natural oil covering the skin, the sebum, especially present in some species such as sheep, appears to maintain the water-holding capacity of the epidermis but has no appreciable role in retarding the penetration of xenobiotics. Disruption of the stratum corneum removes all but a superficial deterrent to penetration. The dermis is a highly vascular area, providing ready access for drug distribution once the epithelial barrier has been passed. The blood supply in the dermis is under complex, interacting neural and local humoral influences whose temperature-regulating function can have an effect on distribution by altering blood supply to this area. The absorption of a chemical possessing vasoactive properties would be affected through its action on the dermal vasculature; vasoconstriction would retard absorption and increase the size of a dermal depot, while vasodilation may enhance absorption and minimize any local dermal depot formation. The appendages of the skin are found in the dermis and extend through the epidermis. The primary appendages are the sweat glands (eccrine and apocrine), hair, and sebaceous glands, all of which show great interspecies and interregional variability. Since these structures extend to the outer surface, they potentially play a role in the penetration of certain compounds. From the perspective of pharmacokinetic models of transdermal and topical drug delivery systems, there are significant differences from other routes of administration (e.g., oral, injection) as to what constitutes a dose. For most exposures, the concentration applied to the surface of the skin exceeds the absorption capacity. However, for therapeutic transdermal patches with a fixed concentration of the drug and rate-controlled release properties, it is the contact surface area that more accurately reflects dose and thus dose is expressed not in mg/kg, but mg/cm2 of dosing area. This surface area dependence also holds for any topical application even if absorption capacity is superseded. Yet, another source of nonlinearity results secondary to the effects of occlusive (water-impermeable) drug vehicles or patches. As the skin hydrates, a threshold is reached where transdermal flux dramatically increases (approximately 80% relative humidity). When the skin becomes completely hydrated under occlusive conditions, flux can be dramatically increased. Therefore, dose alone is often not a sufficient metric to describe topical doses, and application method and surface area become controlling factors. Anatomically, percutaneous absorption might occur through several routes. The current consensus is that the majority of nonionized, lipid-soluble toxicants appear to move through the intercellular lipid pathway between the cells of the stratum corneum, the rate-limiting barrier of the skin. Very small and/or polar molecules appear to have more favorable penetration through appendages or other diffusion shunts, but only a small fraction of drugs are represented by these molecules. Simple diffusion seems to account for penetration through the skin whether by gases, ions, or nonelectrolytes. The rate of percutaneous absorption through this intercellular lipid pathway is correlated to the partition coefficient of the penetrant. This has resulted in numerous studies correlating the extent of percutaneous absorption with a drug’s lipid : water partition coefficient. Some workers further correlated skin penetration to molecular size and other indices of potential interaction between the penetrating molecule and the skin that are not reflected in the partition coefficient. For most purposes, however, dermal penetration is often correlated to partition coefficient. If lipid solubility is too great, compounds that penetrate the stratum corneum may remain there and form a reservoir. Alternatively, penetrated compounds may also form a reservoir in the dermis. For such compounds, slow release from these depots may result in a prolonged absorption half-life. Conditions that alter the composition of the lipid (harsh delipidizing solvents, dietary lipid restrictions, disease) may alter the rate of compound penetration by changing its partitioning behavior. Very similar to the situation with oral absorption previously discussed, the actual utility of a topical drug is a delicate balance between solubility and permeability which topical drug formulators attempt to exploit. Some of these concepts are further discussed in Chapters 5 and 47. Recent studies have demonstrated that the skin may also be responsible for metabolizing topically applied compounds. Both Phase I and II metabolic pathways have been identified. For some compounds, the extent of cutaneous metabolism influences the overall fraction of a topically applied compound that is absorbed, making this process function as an alternate absorption pathway. Cutaneous biotransformation is used to promote the absorption of some topical drugs that normally would not penetrate the skin. Cutaneous metabolism may be important for certain aspects of skin toxicology when nontoxic parent compounds are bioactivated within the epidermis, for example benzo(a)pyrene to an epoxide. Finally, resident bacteria on the surface of the skin may also metabolize topical drugs, as was demonstrated with pentochlorophenol absorption in pig skin dosed in soil with and without antibiotics. This effect is potentiated under warm and wet occlusive dosing conditions that both promote bacterial growth and reduce skin barrier properties. Penetration of drugs through different body regions varies. In humans, generally the rate of penetration of most nonionized toxicants is in the following order: scrotal > forehead > axilla = scalp > back = abdomen > palm and plantar. The palmar and plantar regions are highly cornified producing a much greater thickness that introduces an overall lag time in diffusion. In addition to thickness, the actual size of corneacytes and differences in hair follicle density may affect absorption of more polar molecules. Finally, differences in cutaneous blood flow that have been documented in different body regions may be an additional variable to consider in predicting the rate of percutaneous absorption. These factors are also important in animals, with the area of the inner ear known to be highly permeable and well perfused, thus an excellent site for drug absorption to occur. Although generalizations are tenuous at best, human skin appears to be more impermeable, or at least as impermeable, as the skin of the cat, dog, rat, mouse, or guinea pig. The skin of pigs and some primates serve as useful approximation to human skin, but only after a comparison has been made for each specific substance. The major determinants of species differences are thickness, hair density, lipid composition, and cutaneous blood flow. Soaps and detergents are perhaps the most damaging substances routinely applied to skin. Whereas organic solvents must be applied in high concentrations to damage the skin and increase the penetration of solute through human epidermis, only 1% aqueous solutions of detergents are required to achieve the same effect. For a specific chemical, rate of penetration can be drastically modified by the solvent system used. In transdermal patches, specific chemical enhancers (e.g., solvents such as ethanol; other lipid-interacting moieties) are included in the formulation to reversibly increase skin permeability and enhance drug delivery. Alternatively, drug release is formulated to be rate limiting from the patch system (membranes, microencapsulation, etc.) so that a constant (zero-order) release from the patch occurs, thereby providing controlled drug delivery. Since patches are designed with the permeability properties of specific species in mind, care must be taken when using a patch designed for one species in another. There are a number of topical veterinary drugs routinely used to achieve long-acting systemic therapeutic endpoints in animals. These include the topical pesticides formulated as “spot-ons” and “pour-ons.” These products are further discussed in Chapters 43 and 47 of this text. All of these issues have also been discussed in a comprehensive review of this topic (Riviere and Papich, 2001). Another strategy for transdermal delivery, which has not widely been employed in veterinary medicine, is to overcome the cutaneous barrier by using electrical (iontophoresis) or ultrasonic (phonophoreses) energy, rather than the concentration gradient in diffusion, to drive drugs through the skin. These techniques hold the most promise for delivering peptides and oligonucleotide drugs that now only can be administered by injection. In these cases, dose is based on the surface area of application and the amount of energy required to actively deliver the drug across skin. In iontophoresis, this amounts to a dose being expressed in μAmps/cm2. Formulation factors are also very different since many of the excipients used are also delivered by the applied electrical current in molar proportion to the active drug. Finally, a recent but related strategy is to use very short-duration, high-voltage electrical pulses (electroporation) to reversibly break down the stratum corneum barrier, allowing larger peptides and possibly even small proteins to be systemically delivered. The third major route for systemic exposure to drugs and toxicants is the respiratory system. Since this system’s primary function is gas exchange (O2, CO2), it is always in direct contact with environmental air as an unavoidable part of breathing. A number of toxicants are in gaseous (CO, NO2, formaldehyde), vapor (benzene, CCl4), or aerosol (lead from automobile exhaust, silica, asbestos) forms and are potential candidates for entry via the respiratory system. There are no approved inhalational drugs for use in veterinary medicine. Each mode of inhalational exposure results in a different mechanism of compound absorption and for the purposes of this text, a different definition of dose. These concepts are also discussed In Chapters 11 and 48 of this text. Opportunities for systemic absorption are excellent through the respiratory route since the cells lining the alveoli are very thin and profusely bathed by capillaries. The surface area of the lung is large (50−100 m2), some 50 times the area of the skin. Based on these properties and the diffusion equation presented earlier (Equation 2.1), the large surface area, the small diffusion distance, and high level of blood perfusion maximize the rate and extent of passive absorption driven by gaseous diffusion. The process of respiration involves the movement and exchange of air through several interrelated passages including the nose, mouth, pharynx, trachea, bronchi, and successive smaller airways terminating in the alveoli where gaseous exchange occurs. All of these anatomical modifications protect the internal environment of the air passages from the harsh outside environment by warming and humidifying the inspired air. The passages also provide numerous obstacles and baffles to prevent the inhalation of particulate and aerosol droplets. Thus the absorption of particulate and aerosolized liquids, such as those employed in nebulized drug therapy, is fundamentally different from that of gases. The absorption of such impacted solids and liquids along the respiratory tract has much more in common with oral and topical absorption, with the critical caveat that the precise dose of compound finally available for absorption is very difficult to determine. Great strides have been made in developing aerosol drug delivery devices for human use that take advantage of this mechanism of impaction; however, these may not be transferable to veterinary species since their efficacy is closely related to the geometry and physiology of the human respiratory tract. Another unique aspect of respiratory exposure is the fact that the pulmonary blood circulation is in series with the systemic circulation. Thus, in contrast to cutaneous or oral exposure, compounds absorbed in the lung will enter the oxygenated pulmonary veins that drain to the systemic arterial circulation. Compared to oral administration, this reduces first-pass hepatic metabolism. However, the pulmonary circulation is adept at metabolizing peptides secondary to its role in inactivating peptide hormones. Since the rate of entry of vapor-phase toxicants is controlled by the alveolar ventilation rate, the toxicant is presented to the alveoli in an interrupted fashion whose frequency in humans is equal to the rate of breathing: about 20 times/min. Doses are generally discussed in terms of the partial pressure of the gas in the inspired air. Upon inhalation of a constant tension of a toxic gas, arterial plasma tension of the gas approaches its tension in the expired air. The rate of entry is then determined by the blood solubility of the toxicant. If there is a high blood : gas partition coefficient, a larger amount must be dissolved in the blood to raise the partial pressure. Gases with a high blood : gas partition coefficient require a longer period to approach the same tension in the blood as in inspired air than it takes for less soluble gases. Similarly, a longer period of time is required for blood concentrations of such a gas to be eliminated, thus prolonging detoxification. Another important point to consider in determining how much of an inhaled gas is absorbed into the systemic circulation is the relationship of the fraction of lung ventilated compared to the fraction perfused. Increased perfusion of the lung will favor a more rapid achievement of blood–gas equilibrium. Decreased perfusion will decrease the absorption of toxicants even those that reach the alveoli. Various “ventilation/perfusion mismatches” may alter the amount of an inhaled gas that is systemically absorbed. Similarly, pulmonary diseases that thicken the alveoli or obstruct the airways may also affect overall absorption. The absorption of aerosols and particulates is affected by a number of physiological factors specifically designed to preclude access to the alveoli. The upper respiratory tract, beginning with the nose and continuing down its tubular elements, is a very efficient filtering system for excluding particulate matter (solids, liquid droplets). The parameters of air velocity and directional air changes favor impaction of particles in the upper respiratory system. Particle characteristics such as size, coagulation, sedimentation, electrical charge, and diffusion are important to retention, absorption, or expulsion of airborne particles. In addition to these characteristics, a mucous blanket propelled by ciliary action clears the tract of particles by directing them to the gastrointestinal system (via the glottis) or to the mouth for expectoration. This system is responsible for 80% of toxicant lung clearance. In addition to this mechanism, phagocytosis is very active in the respiratory tract, both coupled to the directed mucosal route and via penetration through interstitial tissues of the lung and migration to the lymph, where phagocytes may remain stored for long periods in lymph nodes. Compared to absorption in the alveoli, absorption through the upper respiratory tract is quantitatively of less importance. However, inhaled toxicants that become deposited on the mucous layer can be absorbed into the myriad of cells lining the respiratory tract and exert a direct toxicological response. This route of exposure is often used to deliver pharmaceutics by aerosol. If a compound is extremely potent, systemic effects may occur. The end result of this extremely efficient filtering mechanism is that most inhaled drugs deposited in nasal or buccal mucous ultimately enter the gastrointestinal tract. This can best be appreciated by examining the respiratory drainages depicted in Figure 2.1. Therefore, the disposition of aerosols and particulates largely mirrors that of orally administered drugs. Nasal administration is a preferred route for many inhalant medications in humans. In these cases, great care is made to deliver aerosols of the specific size for deposition on the nasal mucosa and upper respiratory tract. The bioavailability of these compounds is assessed using the techniques developed for other routes, although a local effect is often desired. The problems with this strategy are the attainment of an accurately delivered dose and the inactivation and binding of administered drug by the thick mucous blanket. Drugs delivered by this route usually have a wide therapeutic window and large safety index. The final point to consider relates to some specific peculiarities of nasal absorption. In the region of the olfactory epithelium, there exists a direct path for inhaled compounds to be absorbed directly into the olfactory neural tissue and central nervous system, thereby bypassing both the systemic circulation and the blood–brain barrier. The mass of drugs involved in this uptake process is very small and thus would not affect a pharmacokinetic analysis. However, this route has obvious toxicological significance and unfortunately has not been carefully studied in veterinary species. In order to complete this discussion of absorption, it is important to realize that there are other extravascular drug administration routes that are often encountered. Relative to pharmacokinetic analysis, these are dealt with in the same fashion as the primary routes discussed above. The important difference is that in all cases, the barrier to absorption is less than that encountered in oral or topical delivery. Second, all of these routes involve an invasive procedure to inject drug into an internal body tissue, thereby bypassing the epithelial barriers of the skin and gastrointestinal tract. The primary therapeutic routes of drug administration are subcutaneous (SC or SQ) and intramuscular (IM). In these cases, the total dose of drug is known and injected into tissue that is well perfused by systemic capillaries that drain into the central venous circulation. Both of these routes as well as intravenous administration are termed parenteral to contrast primarily with oral (enteral) and topical dosing, which are classified as nonparenteral routes of drug administration. A primary difference between these two classes is that parenteral routes bypass all of the body’s defensive mechanisms. Parenteral dosage forms are manufactured under strict guidelines that eliminate microbial and particulate contamination resulting in sterile preparations that must be administered using aseptic techniques. This restriction does apply to oral or topical dosage forms. As with all methods of drug administration, there are numerous variables associated with SC and IM dosing that can be conveniently classified into pharmaceutical and biological categories. Finally, there are other occasional routes of drug administration employed that require absorption for activity. Administration of drugs by intraperitoneal injection is often used in toxicology studies in rodents since larger volumes can be administered. Peritoneal absorption is very efficient, provided adequate “mixing” of the injection with the peritoneal fluid is achieved. The majority of drug absorbed after interperitoneal administration enters the portal vein and thus may undergo first-pass hepatic metabolism. The disposition of intraperitoneal drug thus mirrors oral administration. Some drugs are administered by conjunctival, intravaginal, or intramammary routes. In these cases achievement of effective systemic concentrations are often not required for what is an essentially local therapeutic effect. Prolonged absorption from these sites may result in persistent tissue residues in food-producing animals if the analytical sensitivity of the monitoring assay is sufficiently low. The systemic absorption of these dosage forms is quantitated using procedures identical to those employed for other routes of administration. The final topic to consider with absorption is the assessment of the extent and rate of absorption after oral, topical, or inhalational drug administration. The extent of drug absorption is defined as absolute systemic availability and is denoted in pharmacokinetic equations as the fraction of an applied dose absorbed into the body (F). Although this topic will also be discussed extensively in Chapter 3, it is important and convenient at this juncture to introduce the basic concepts so as to complete the discussion of drug absorption. If one is estimating the extent of drug absorption by measuring the resultant concentrations in either blood or excreta, one must have an estimate of how much drug normally would be found if the entire dose were absorbed. To estimate this, an intravenous dose is required since this is the only route of administration that guarantees that 100% of the dose is systemically available (F = 1.0) and the pattern of disposition and metabolism can be quantitated. Parameters used to measure systemic availability are thus calculated as a ratio relative to the intravenous dose. For most therapeutic drug studies, systemic absorption is assessed by measuring blood concentrations. The amount of drug collected after administration by the route under study is divided by that collected after intravenous administration. When drug concentrations in blood (or serum or plasma) are assayed, total absorption is assessed by measuring the area under the concentration–time curve (AUC) using the trapezoidal method. This is a geometrical technique that breaks the AUC into corresponding trapezoids based on the number of samples assayed. The terminal area beyond the last data point (a triangle) is estimated and added together with the previous trapezoidal areas. Absolute systemic availability then is calculated as in Equation 2.4:
Absorption, Distribution, Metabolism, and Elimination
An Overview of Drug Disposition
Drug Passage Across Membranes
Absorption
Gastrointestinal Absorption
Disintegration, Dissolution, Diffusion, and Other Transport Phenomenon
Enterohepatic Recycling and Coprophagy
Species Effects on Gastrointestinal Transit Time and Food Interactions
First-Pass Metabolism
Formulation Factors
Disintegration
Excipients
Compaction pressure
Enteric coatings, capsules
Homogeneity
Dissolution
Particle size/surface area
Binding
Local pH, buffers
Boundary layers
Barrier diffusion
Solubility
Transit time
Topical and Percutaneous Absorption
The Stratum Corneum Barrier
Dermis and Appendages
Topical Drug Delivery and the Definition of Dose
Pathways for Dermal Absorption
Variations in Species and Body Region
Factors that Modulate Absorption
Respiratory Absorption
Vapors and Gases
Aerosols and Particulates
Other Routes of Administration
Bioavailability
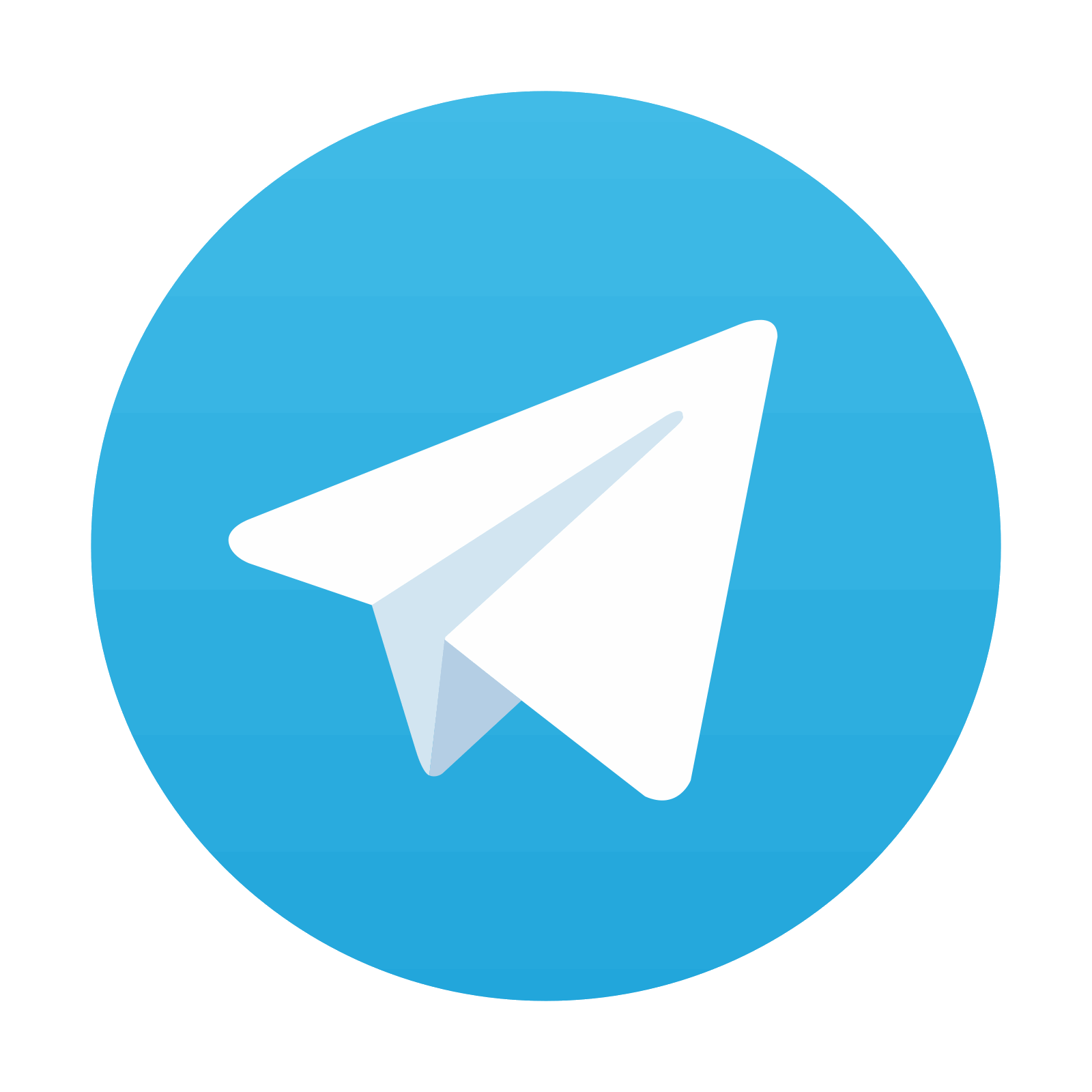
Stay updated, free articles. Join our Telegram channel
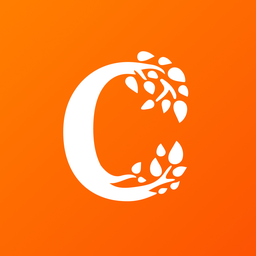
Full access? Get Clinical Tree
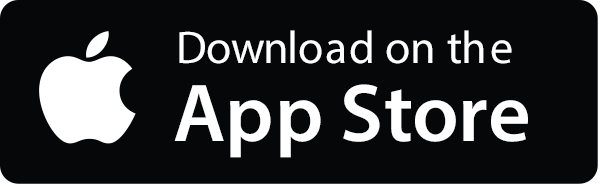
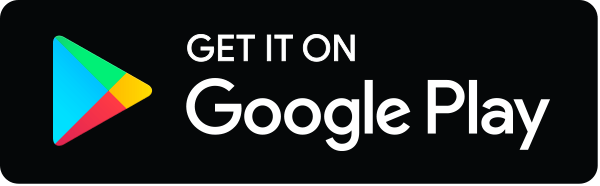