Normal urine from dogs and cats contains very little, if any, protein (mg/dL) when measured by conventional urine chemistry dipstrip methods. Albumin is the predominant protein detected in urine from normal dogs [1] and cats as well as those with renal disease [2]. Some dogs [3] and cats with chronic kidney disease (CKD) have predominantly nonalbumin proteinuria characteristic of tubular proteinuria. Small quantities of urinary protein can be detected in urine from healthy individuals using more sensitive analytical methods such as sulfosalicylic acid (SSA), urinary protein‐to‐creatinine ratio (UPC), microalbuminuria (MA), and urinary protein electrophoresis. Plasma proteins <40 kD are considered low‐molecular‐weight (LMW) proteins that easily cross the glomerular barrier. Intermediate‐weight proteins that are approximately the size of albumin at 65 kD do not readily cross the glomerulus based on size and charge, whereas high‐molecular‐weight (HMW) proteins >100 kD do not cross the normal glomerular barrier at all. Immunoglobulin G (IgG), immunoglobulin M (IgM), and immunoglobulin A (IgA) are examples of HMW proteins that do not cross the glomerulus unless it is damaged [4–6]. “Proteinuria” is the general term used to describe the detection of an abnormally high quantity of protein in the urine due to a pathological condition. Proteinuria can be caused by prerenal, postrenal, and primary renal disorders (Table 7.1). Trauma or contamination from the method of urine collection must be considered as a possibility of adding plasma proteins along with red blood cells (RBC) to urine (catheterization, cystocentesis, or voiding). Proteinuria may indicate a pathologic process anywhere in the urinary tract (i.e. kidney, ureter, urinary bladder, urethra) in addition to that from iatrogenic trauma or contamination from the genital tract. The finding of proteinuria provides a diagnostic clue for disorders of the upper and lower urinary tract. The findings from urine specific gravity (USG) and urinary sediment microscopy are important components of the complete urinalysis that help determine the importance of the proteinuria and its likely origin. A combination of findings from history, physical examination, imaging, hematology, routine serum biochemistry, and urinalysis may be needed to determine the origin of the proteinuria. Sometimes, the cause of the proteinuria is readily identified in animals with inflammatory lower urinary tract disease based on history and physical exam (e.g. urinary tract infection [UTI], urolithiasis, and neoplastic masses). Renal biopsy, with special techniques of light microscopy, electron microscopy (EM), and immunofluorescent antibody (IFA), is needed to determine with certainty the underlying lesions resulting in renal proteinuria [26, 27] in patients in which prerenal and postrenal causes have been eliminated as a differential consideration. The concentration of proteins in urine leaving the kidney in health depends on the extent that protein was filtered across the glomerulus, the extent of tubular reabsorption, any additions of protein from tubular secretion of Tamm–Horsfall protein (THP) or from cellular or intracellular contents [6, 16, 20], and the degree of water removed by the tubules during the process of urine concentration. The glomerulus performs the first step in urine formation (as reviewed in Chapter 1, Figure 1.10). A detailed review of the molecular structures and functions of the glomerular basement membrane (GBM), including GBM assembly, maintenance, and repair, is available for the interested reader [28]. The glomerular capillary membrane functions to readily allow an ultrafiltrate of plasma (water, nonprotein bound electrolytes, and small‐molecular‐weight waste products) to appear in Bowman’s space, while restricting the passage of cells and large‐molecular‐weight plasma proteins across the glomerulus at the same time. The glomerulus displays a sophisticated system of structural and functional effects that limit the entry of larger plasma proteins and cells into Bowman’s space. Normal glomerular barrier function, sometimes called permselectivity, involves resistance to the passage of molecules based on their size and charge. The unique arrangement and function of the glomerular endothelium, endothelial fenestrae, basement membrane (lamina rara interna, lamina densa, and lamina rara externa), podocyte foot processes, slit pore membranes, and podocyte cell bodies provide an integrated system that maintains normal glomerular barrier function and glomerular filtration (Figures 7.1 and 7.2). Type IV collagen, laminin, nidogen, and perlecan, a proteoglycan, are the major protein constituents of GBM [29]. Collagen IV is the main structural component of the framework of the GBM, which is important for the permselective barrier [30, 31]. The anionic charge of the GBM is important in hindering the filtration of negatively charged plasma proteins [31]. A large amount of protein enters the glomerular capillaries (6–8 g/dL) from the systemic circulation, but very little passes through the glomerulus into the urinary space and then into the proximal tubule for further processing. Albumin is the predominant circulating protein (3–4 g/dL) that enters the glomerulus. Albumin constitutes 40–60% of the proteins in normal urine due to incomplete proximal tubular reabsorption of the small amount of albumin filtered from plasma across the glomerulus into tubular fluid [32, 33]. The remaining 40–60% of the protein in normal urine originates from protein secreted by the distal tubule and collecting duct (THP), as well as protein contributed by the urothelium of the lower urinary tract and genital tract [33, 34]. Normal glomerular barrier function impedes the passage of proteins across the glomerulus due to the unique interaction and function of the endothelial cells, basement membrane, and the podocytes that comprise the glomerulus. The podocyte is important in the development of the glomerular endothelium and maintenance of its fenestrations [31]. The morphology and function of the podocyte is largely determined by its complex cytoskeleton and interactions with proteins in the slit pore diaphragm (Figure 7.3) [35, 36]. The slit pore membrane is a zipper‐like structure with a constant width [31]. Podocytes help to control shape, structure, and spatial orientation of the glomerulus through contractility, which is important in maintenance of glomerular filtration rate (GFR) [35]. Table 7.1 Classification of proteinuria based on site or mechanism. Source: Vaden and Elliott [7]; Grauer [8]; DiBartola [9]; Grauer [2]; Grauer [10]; Harley and Langston [11]; Whittemore [12]; Whittemore [13]; Lees et al. [14]; Langston [15]; Lamb and Jones [16]; Harrison et al. [17]; Aguado et al. [18]; Osserman and Lawlor [19]; Delaney and Lamb [20]; Summers [21]; Hedgespeth and Harrell [22]; Benzing [23]; Albright [24]; Norden et al. [25]; MW – molecular weight, MA – microalbuminuria, LUT – lower urinary tract, UPC – urinary protein to urinary creatinine ratio, TVT – transmissible venereal tumor, AKI – acute kidney injury, ATN – acute tubular necrosis, ARF – acute renal failure. FIGURE 7.1 Schematic representation of the glomerulus as seen in transverse section. The urinary space lies outside the podocytes where ultrafiltrate accumulates on its way to the proximal tubules for further processing. The basic layers of the glomerulus are shown (from within the capillary lumen to the urinary space) as the endothelial cells and their fenestrations, basement membrane of the endothelial cells and podocytes, podocytes (also called visceral epithelium) and their foot processes. The mesangium consists of mesangial cells and mesangial matrix. Note that there is only one layer of basement membrane around the mesangium. Mesangial cells can proliferate and lay down more mesangial matrix during some kidney diseases. Mesangial cells also have contractile properties that can alter the available surface area for glomerular filtration in health and disease. The podocyte provides cytoskeletal support for normal glomerular architecture. Not shown is the slit pore membrane between the podocyte feet. Proper slit pore function is necessary to retard passage of plasma proteins across the glomerulus. Glomerular origin proteinuria can occur when any of these anatomical sites are disturbed. Source: Illustration by Tim Vojt, reproduced with the permission of The Ohio State University. Nephrin, the first slit pore protein to be identified [31], appears to be the most important molecule within the slit pore membrane for the maintenance of normal podocyte function [37]. Nephrin is a major component of the 40‐nm slit diaphragm protein complex between adjacent podocyte foot processes. Nephrin and Neph1 are transmembrane proteins with extracellular domains that form the slit membrane. They also importantly regulate the actin cytoskeleton, which controls the shape and ultrastructure of the podocyte and foot process apposition to the GBM. Nephrin and Neph1 interact with the membrane protein podocin, which influences the expression of transient receptor potential cation channel 6 as a mechanosensitive channel in the slit diaphragm. Genetic or acquired defects in any proteins comprising the slit membrane or podocyte membrane consistently result in proteinuria [23, 36]. Endothelial cells of the glomerulus have numerous fenestrated openings of 70–100 nm diameter [31]. The normal glomerulus does not generally allow plasma proteins with a size equal to or greater than albumin (MW 67 kD and 3.5 nm diameter) to cross into Bowman’s space and then into tubular fluid [16]. Very small molecules, such as inulin, creatinine, urea, glucose, and nonprotein bound electrolytes, are freely filtered across the glomerulus into the urinary space, as is water. Normal glomerular barrier function opposes the passage of negatively charged plasma proteins as the various structural layers of the glomerulus are negatively charged. The surface of endothelial cells has a negative electric charge because of the presence of glycoproteins, glycosaminoglycans, and the glycocalyx [28,38–40]. This negative charge acts as a barrier to the interaction of anionic proteins such as albumin with endothelial cells. Normally, circulating negatively charged albumin is repelled from the endothelial surface due to electrical repulsion, decreasing the permeability of albumin. Larger molecules that are negatively charged and globular in shape (such as albumin and IgG) will not readily cross the normal glomerulus and are retained within the circulation [16, 20]. FIGURE 7.2 Schematic three‐dimensional view of the glomerulus as would be observed during scanning electron microscopy. The filtration barrier consists of capillary endothelium, glomerular basement membrane, and visceral epithelial cells (podocytes). The glomerular endothelium is highly fenestrated (relatively unique to the kidney), and these openings of 50–100 nm in diameter are larger than those in systemic capillaries. These openings restrict the passage of cells (WBC, RBC, and platelets) based on their large size. The endothelium and basement membranes are coated with negatively charged glycocalyx (sialoglycoproteins) that repel plasma proteins that have a net negative charge. A breakdown in this coating is associated with transglomerular passage of plasma proteins. Podocytes with major and minor foot processes are shown in relationship to the glomerular basement membrane. The slit pore (not shown) is between the minor foot processes. Source: Illustration by Tim Vojt, reproduced with permission of The Ohio State University. The normal GBM and podocytes counteract luminal pressure from within the glomerular capillary. The interdigitating podocyte foot processes normally compress the gel‐like composition of the GBM. Buttressing force from the podocytes that compress the GBM helps to maintain the shape of the GBM and restrict the passage of macromolecules such as albumin across the GBM (permselectivity). As podocytes are injured, the buttressing forces from the podocyte foot processes are decreased, which results in less compression of the GBM. This results in a shape change of the GBM and increased permeability to molecules of the size of albumin and larger across the GBM. Injury to podocytes also reduces the length of the slit diaphragm, which reduces the surface area available for glomerular filtration of water and small molecules at the same time that increased permeability to proteins happens. This reduction in GFR is counteracted to some degree by the effect of angiotensin‐II (AG‐II) to increase the vasoconstriction of the efferent arteriole, which increases GFR. This “compensatory” increase in GFR is associated with increased glomerular capillary pressure that is no longer able to be counteracted (buttressed) by the diseased podocytes, resulting in more proteinuria. Since podocytes are mechanically sensitive, an increased GFR in single nephrons (hyperfiltration) with decreased surface area exposes the podocytes to more damage or podocyte detachment from sheering stress that occurs from increased fluid flow across the glomerulus [23, 36]. Though podocytes exert a pivotal role in maintaining glomerular barrier integrity, alterations in the structure and function of the GBM or glomerular endothelial cells also result in albuminuria, so it is important to consider all three of these possibilities as mechanisms resulting in glomerular proteinuria [23]. FIGURE 7.3 Podocytes in the glomerulus as seen on scanning electron microscopy, looking at the glomerulus from the urinary space in Bowman’s capsule – 800×. Podocytes have narrow cell extensions (processes) that give rise to secondary extensions called pedicels. The podocytes completely surround the capillary system. CB – cell body; MP – major foot process; SP‐ secondary foot process; FP – finely interdigitating foot process. Source: Dennis Kunkel Microscopy / Science Photo Library. Once the glomerular ultrafiltrate reaches the proximal tubule, it undergoes changes in composition due to water and solute reabsorption. The final urinary excretion of proteins largely depends on the interaction of protein with proximal tubular epithelial cells. The proximal tubular epithelial cells have a prominent layer of microvilli, which is the site for megalin‐receptor‐mediated endocytosis of negatively charged proteins. The megalin receptor and its interaction with cubulin is important in the proximal tubular reabsorption of albumin and some other molecules like vitamin‐D‐binding protein (VDBP) [6, 20, 38,41–44]. Albumin binds to this specific membrane receptor complex and is internalized by membrane vesicles [45–47]. These endocytic vesicles fuse with lysosomes that degrade the protein into amino acids, which are released at the basolateral surface of the tubular cell into the interstitium and peritubular capillaries [20]. The very small amount of plasma albumin that was sieved across the normal glomerulus into tubular fluid undergoes 99% reabsorption by the proximal tubule in health. The plasma proteins of lower molecular weight that freely crossed the glomerular filter are also reabsorbed by normal tubules [16, 20]. Tamm–Horsfall mucoprotein (THP, Tamm–Horsfall glycoprotein [THG], uromodulin) is an HMW globulin (90–130 kD) produced by the distal tubules and collecting ducts as well as the thick ascending limb of the loop of Henle [3, 32, 48, 49]. The tubular secretion of THP or THG by the thick ascending limb of Henle’s loop and the early distal tubule contribute to the small amount of protein content in normal urine [3, 16, 20, 50]. THP was found in normal dog urine at a concentration of 0.5–1.0 mg/dL, similar to that in humans in one study [32], and at these low levels, it is not detected by conventional semiquantitative urinary tests. The median urinary THP (ng/mL measured by ELISA; 2.5–500 ng/mL standard curve) to urinary creatinine (mg/dL) × 10−2 ratio was 101 (58–172) in healthy dogs and 0 (0–88) in dogs with CKD of one study [51]. The ratio of THP (measured by quantitative Western Blot) to urinary creatinine was also reduced in CKD dogs with moderate‐to‐severe azotemia and proteinuria of another study. The median THP‐to‐urinary‐creatinine ratio in nonazotemic and nonproteinuric dogs was 15.2 mg/g (9.62–65.2) [49]. Decreased urinary THP was also demonstrated in another study of dogs with CKD [3]. Proteinuria may be classified as prerenal, renal (functional renal, pathologic tubular, pathologic interstitial, or pathologic glomerular), or postrenal in etiology (Figure 7.4 and Table 7.1 [2,7–16]. Proteins in urine can appear following the increased filtration of plasma proteins across the glomerulus, the failure of proximal tubular epithelial cells to reabsorb protein, the addition of proteins from tubulointerstitial inflammation and degeneration, and the addition of plasma proteins to urine from anatomical sites below the kidney. When postrenal proteinuria has been excluded, the presence of medium‐to‐high‐molecular‐weight proteins (such as albumin) in urine usually indicates the loss of normal glomerular barrier function. For renal origin proteins with LMW, the defect causing proteinuria is likely related to decreased function of proximal renal tubular cells. Mixed patterns of renal proteinuria can include a varying degree of glomerular and tubular origin proteinuria. FIGURE 7.4 Categories of Proteinuria. The detection of proteinuria by itself does not determine where it originated. The first decision is whether the proteinuria is from the urinary tract or from nonurinary tract sources. Prerenal proteinuria is uncommon. Genital tract sources for proteinuria occasionally occur during prostatic or uterine/vaginal disease. Postrenal sources of proteinuria from lower urinary tract disorders are very common. Renal proteinuria that is persistent is pathological and most commonly occurs due to glomerular diseases. Prerenal proteinuria is uncommon in dogs and cats. The glomerular barrier function is normal. It occurs following hemoglobinemia during systemic hemolysis, myoglobinemia from traumatized muscle, and production of light chains (Bence Jones proteins) associated with multiple myeloma or other paraproteinemia. These small‐molecular‐weight proteins readily filter through the normal glomerulus from plasma to enter the urine [2, 9, 14]. Prerenal proteinuria can be considered an overload or overflow of LMW molecules not normally present in plasma that are freely filtered across the glomerulus. These proteins enter tubular fluid in an amount that exceeds the ability of tubules to reabsorb the particular protein [16]. There are no changes in the urinary tract anatomy, and the urinary sediment contains very few elements supporting inflammation. Granular casts containing hemoglobin, myoglobin, or Bence Jones proteins can sometimes form. Acute kidney injury (AKI) can occur in these conditions, which would add a component of tubular proteinuria and possibly cellular casts (see below) to the prerenal proteinuria. Pigmented plasma may be present due to binding of hemoglobin to a carrier, whereas myoglobinemia is not associated with pigment in the plasma as there is no plasma carrier for myoglobin [52]. A screening test to distinguish myoglobin from hemoglobin uses ammonium sulfate to precipitate and remove hemoglobin, but the definitive measurement of myoglobin requires the use of immunochemical methods [16]. Bence Jones proteins are suspected in urine when the heat test is positive for precipitation [53], but urinary electrophoresis with immunofixation is considered definitive [16]. Postrenal proteinuria is the most common type of proteinuria in veterinary medicine [2, 10]. In postrenal proteinuria, glomerular barrier function is normal. Protein enters the lower urinary tract from the circulation following hemorrhage or during inflammation at anatomic sites below the kidneys, including the lower urinary and genital tracts. The history may include signs of lower urinary tract urgency (dysuria, stranguria, pollakiuria). Results from physical examination and imaging often reveal structural changes to the lower urinary tract anatomy. Urinary sediment is abnormal with varying combinations of hematuria, pyuria, bacteriuria, epitheliuria, and crystalluria. The most common causes for postrenal proteinuria include trauma, obstruction, urinary infection, inflammation, neoplasia, or urolithiasis. Renal proteinuria occurs when plasma proteins excessively cross the glomerulus, the proximal renal tubules fail to reabsorb filtered proteins, or protein is added to the urine as a result of tubulointerstitial bleeding and inflammation. Renal proteinuria develops as a consequence of many primary renal diseases and also when a systemic disorder secondarily involves the kidneys. Prerenal and postrenal sources of proteinuria must be ruled out before renal proteinuria can be diagnosed with certainty. Renal functional or physiological proteinuria is attributed to changes in glomerular vascular tone without structural renal disease that result in albuminuria. Fever, seizures, extremes of heat and cold, renal congestion, and severe stress can lead to this type of proteinuria. Functional proteinuria is transient and disappears when the underlying condition resolves as there is no permanent change to the glomerulus [2, 9]. Pathological renal proteinuria can originate from glomerular, tubular, or tubulointerstitial causes (Table 7.2). Primary glomerular diseases include glomerulonephritis (GN), amyloidosis, glomerulosclerosis, and hereditary nephropathy (HN). Secondary glomerular barrier dysfunction can occur in systemic diseases characterized by chronic antigen excess and antibody formation, endocrinopathy, and during critical illness. Renal tubular proteinuria can occur during AKI and in those with congenital or acquired Fanconi syndrome (FS) (see Chapter 5 and Table 7.2). Though glucosuria is often the major initial feature discovered in the generalized proximal tubular defect in FS, renal proteinuria can also be found. LMW proteinuria following the failure of proximal tubules to reabsorb freely filtered plasma proteins is commonly documented. HMW proteinuria that includes albumin can also be identified in humans with FS. LMW tubular protein markers accounted for about 50% and albumin accounted for about 30% of the proteins in urine in one report of FS in humans. Proteinuria was attributed to the failure of receptor‐mediated endocytic reabsorption by proximal tubules to reclaim the small quantities of these proteins that are normally present in proximal tubular fluid [25]. Though aminoaciduria has been well characterized in FS in dogs, the magnitude and nature of proteinuria as to tubular or glomerular origin has not [90, 91]. Angiotensin‐converting enzyme inhibitor (ACE‐I) treatment reduced the magnitude of albuminuria in children with FS associated with cystinosis but alpha‐1 microglobulinuria did not change [92]. Abnormal glomerular barrier function is the most common mechanism leading to pathological renal proteinuria. Interactions between glomerular capillary pressure, the GBM, and podocytes importantly determine glomerular permeability to circulating proteins including albumin. Damaged podocytes result in proteinuria (albuminuria) as the podocyte assumes a simplified architecture characterized as foot‐process effacement (fusion of foot processes) and a shortening of the slit pore diaphragm; this early lesion is potentially reversible. Podocyte loss due to detachment or necrosis is irreversible since podocytes are postmitotic cells with limited ability to regenerate themselves, which results in fibrosis. Most types of podocyte injury are acquired, and many are created by immune injury to various podocyte target antigens in human medicine. Podocyte injury is associated with changes in the GBM in most instances. Decreased GFR occurs in renal diseases, in which the glomerular surface area available for filtration is reduced or when intrinsic glomerular permeability is decreased. The protection of the podocyte as a means to prevent the progression of CKD was described as a main treatment goal in human medicine [23, 36]. Experimentally injured podocytes allow molecules of the size of albumin and larger to enter the GBM that are then taken up into the podocytes. Some circulating molecules traverse the GBM but do not pass through the slit diaphragm leading to the notion that the GBM is a coarse filter and the slit diaphragm is a fine filter. It appears that podocyte injury is central to all proteinuric renal diseases [23]. A variety of systemic and primary renal disease processes can decrease glomerular barrier integrity so that there is less resistance to the passage of plasma proteins (mostly albumin early in the disease) into Bowman’s space. The hallmark of glomerular‐origin proteinuria (regardless of specific disease) is the documentation of excess urinary protein in association with a noninflammatory urinary sediment (≤10 RBC/high‐power field (HPF), ≤5 white blood cells (WBC)/HPF, <2 nonsquamous epithelial cells/HPF). Glomerular proteinuria can be associated with increased excretion of casts, especially hyaline casts and sometimes a few granular casts in which filtered proteins precipitate as granules (see Chapter 6 for more details). It should be noted that newer thinking suggests that lower urinary tract inflammation or hemorrhage contributes less to the measurement of urinary proteins than has been traditionally considered to be important [93, 94]. Primary glomerular disease with heavy renal proteinuria is far less common in cats than in dogs [26, 54, 95]. CKD in cats is generally idiopathic, and tubulointerstitial lesions with fibrosis predominate. Episodic renal hypoxia has been speculated to play an important role in the development and progression of these types of CKD lesions in cats [95]. In 58 cats with renal proteinuria, the origin of the proteinuria was glomerular in 42, tubular in 11, and other or both in 5 cats [96]. Immune‐complex glomerulonephritis (ICGN) (31 of 42 cats) was the most common diagnosis in cats with glomerular proteinuria; other glomerular diseases were diagnosed in 11 cats. UPC was borderline increased (0.2–0.3, median 0.26) in four cats with tubulointerstitial disease, and significantly increased (1.0–5.8, median 2.2) in seven cats with tubulointerstitial disease. In 42 cats with protein‐losing nephropathy (), median UPC was 8.38 (0.45–30). The median UPC was significantly lower at 6.5 (1.81–13.3) in cats with ICGN compared to cats with other glomerular diseases that exhibited a median UPC of 14.5 (10.1–30). Interestingly, 4 of 12 cats with tubulointerstitial disease had a UPC > 2.0 and 5 of 43 cats with primary glomerular lesions had a UPC < 2.0. This demonstrates that the general notion that a UPC > 2.0 is associated with primary glomerular disease is not absolute [96]. Table 7.2 Differential diagnoses associated with renal proteinuria. Refer to the text for more detailed information about specific differentials, but not all disorders listed in the table are discussed in the text. References for several of the causes of renal proteinuria are provided here (Harley and Langston [11]; Roura [54]; Syme and Elliott [55]; Vaden and Grauer [56]; Vaden [57]; DiBartola et al. [58]; Dibartola and Westropp [59]; Cianciolo et al. [27]; Schneider [60]; Cianciolo et al. [61]; Crisi et al. [62]; Wright and Cornwell [63]; Crowell and Barsanti [64]; Nash and Wright [65]; Zeugswetter et al. [66]; Bennett and Nash [67]; Johnson and Mackin [68]; Johnson and Mackin [69]; Furrow et al. [70]; Smith et al. [71]; Crowell and Leininger [72]; Vilafranca et al. [73]; Aresu et al. [74]; Gori et al. [75]; Paltrinieri et al. [76]; Ibba et al. [77]; Winiarczyk et al. [78]; Brown et al. [79]; Backlund et al. [80]; Grauer et al. [81]; Grauer et al. [82]; Page et al. [83]; Dobenecker et al. [84]; Alexander et al. [85]; Laflamme et al. [86]; Grauer et al. [87]; Clinkenbeard et al. [88]; Yang [89]; Norden et al. [25]; Bovee et al. [90]; Yearley et al. [91]). In another study of 68 cats with proteinuric CKD, slightly greater than half the cats had ICGN (37/68); the remaining cats were classified as having non‐ICGN. Membranous or membranoproliferative glomerular lesions were those most commonly described in those with ICGN. The mean UPC at the diagnosis of ICGN was 7 ± 3.2 (median: 2.6; 2.4–18.6). The magnitude of UPC was lower in cats with non‐ICGN compared to those with ICGN. Mean UPC in the non‐ICGN cats increased with the severity of the tubulointerstitial lesions (mean range of 1.5–3.1). A UPC > 3.8 was considered the best cutoff point to distinguish those with ICGN from those with other causes of glomerular disease in this study [97]. In one study from the USA, 501 dogs with renal proteinuria suspected to have GN underwent renal biopsy (Figure 7.5). ICGN was the diagnosis in 48.1% of these dogs, 20.6% had primary glomerulosclerosis, 15.2% had amyloidosis, 9% had nonimmune‐complex glomerulopathy, 4.8% had nonimmune‐complex nephropathy, and 2.4% had primary tubulointerstitial disease [27]. An accurate diagnosis required analysis using light, immunofluorescent, and EM [27, 60, 61]. In a study from mainland Europe, 162 renal biopsies from dogs suspected to have glomerular disease were evaluated by light and EM. ICGN was the final diagnosis in 50.6%, 36.4% as nonimmune‐complex‐mediated GN, and 13% nonspecified renal lesions. The number of cases with the diagnosis of ICGN increased substantially over that made by light microscopy alone when results from EM were used as the gold standard for the presence of immune complexes [74]. The diagnosis of ICGN was less frequent in 62 dogs with renal proteinuria from the UK compared to those in the USA or mainland Europe. ICGN occurred in 27% of the dogs, glomerulosclerosis in 35%, nonspecific glomerulopathy in 13%, amyloidosis in 11%, and unspecified renal lesion in 13% following evaluation by light, immunofluorescent, and EM [98]. Renal proteinuria develops long before overt loss of excretory renal function is identified in humans with a variety of glomerular diseases [99]. Renal proteinuria is also observed before the onset of azotemic CKD in dogs and cats in general [10] and in dogs with X‐linked HN [100]. Clinical studies have shown various degrees of proteinuria or albuminuria (none, borderline, overt) at the time of diagnosis of CKD in dogs and cats, but the temporal role of proteinuria preceding the diagnosis and its role in development of CKD has not been determined in most forms of CKD. FIGURE 7.5 Membranoproliferative glomerulonephritis. Renal biopsy by light microscopy 40X PAS, consensus report from the International Veterinary Renal Pathology Service (IVRPS). Note thickened glomerular capillary loops and glomerular adhesion between visceral and parietal epithelium. This is from a three‐year‐old Boxer with azotemic CKD and PLN. The dog had a two‐to‐three‐week history of anorexia, vomiting and weight loss. She had mild hypoalbuminemia (2.2 g/dL), serum creatinine of 3.9 mg/dL, and UPC of 12.7 in urine with an inactive sediment. Mildly hyperechoic kidneys with mild pyelectasia were seen on ultrasonography. Serologic testing for heartworms, leptospirosis, and tick‐borne diseases were negative. There were no body effusions or edema despite the marked proteinuria and hypoalbuminemia. Systolic blood pressure was 150–160 mmHg initially, but occasionally, it was 180–190 mmHg. A variety of immunological and nonimmunological mechanisms can be important in the development of glomerular proteinuria [31, 99, 101]. All layers of the glomerulus work together in an integrated way to maintain glomerular barrier function, which normally prevents proteinuria. Consequently, proteinuria is an expected consequence of damage or change in function for any component or layer of the glomerulus [38]. A net negative charge of the endothelial cells, GBM, and the visceral epithelial cells (podocytes) is important in thwarting transglomerular passage of negatively charged plasma proteins [99, 102]. A loss of this net negative charge can occur during many diseases of the glomerulus, which then favors the development of glomerular proteinuria (albuminuria) [102]. Loss of the negative charge of the glycocalyx also exposes the podocytes to damaging effects of albumin and other macromolecules [101]. The downregulation of megalin receptor expression occurs when tubular fluid albumin content is high, and this can contribute to increased tubular cell apoptosis [20]. The capacity of the proximal tubules to reabsorb proteins from the tubular fluid is also limited as receptor binding can become saturated during tubular fluid protein overload. Tmax for protein reabsorption by the proximal tubule can be exceeded when diseases lead to an increase of filtered proteins presented for binding to the proximal tubule. A vicious cycle of nephron loss and development of intraglomerular hypertension in surviving adapting nephrons leads to proteinuria during CKD [102–104]. Additional adaptations include glomerular hypertrophy, increased single‐nephron GFR, and mesangial matrix expansion that contribute to proteinuria in dogs and cats [105, 106]. Protein in urine can be measured by qualitative, semiquantitative, or quantitative methods. The gold standard for the evaluation of proteinuria is the quantitative measurement of total protein and or albumin from an accurately timed and collected 24‐hour urine specimen. Quantitative results are reported in total mg/day or mg/kg/day [9, 16]. A 24‐hour urine collection is uncommonly performed in clinical practice, due to the difficulty in accurately collecting all urine during this time and from the effects of stress from hospitalization needed for the procedure [16]. It is more difficult to measure total proteins in urine than in serum due to low concentration of urinary proteins and high concentrations of potentially interfering substances in urine, which affect the precision and accuracy of these methods. Methods to measure total proteins vary in their sensitivity and specificity to detect all proteins. Most methods to measure total protein favor the detection of albumin over globulins and nonalbumin proteins [16]. The measurement of urinary protein on the chemistry dipstrip is the most common method to initially evaluate proteinuria (see Chapter 5). The finding of dipstrip positive proteinuria can be detected in those in which no urinary disease was originally suspected, during the screening of patients with known CKD or AKI, or in those with lower urinary tract signs (postrenal proteinuria). The evaluation of proteinuria with other methods such as SSA, UPC, or MA should be used to confirm and further characterize the presence or absence of renal proteinuria. Renal proteinuria provides diagnostic information as supporting the presence of renal disease, but it also serves as a prognostic indicator since survival of dogs and cats with CKD is related to the degree of renal proteinuria. The evaluation of protein by dipstrip, SSA, UPC, and MA at the same time on the same urine sample has the potential to provide more clinically useful information than from any of these tests individually, but this is not yet considered standard of approach. Individual proteins in urine are best measured by immunoassay [16], but this is beyond the scope of this chapter. The cellulose pad on the dipstrip contains the inert base tetrabromphenol blue (3′,3″,5′,5″‐tetrachlorophenol‐3,4,5,6‐tetrabromosulfophthalein) buffered with citrate to a pH of 3.0 [16, 107, 108]. The color that develops in the presence of protein is based on the “protein error of indicators” [16, 109]. The protein error of indicators refers to the ability of protein to change the color of the acid–base indicator (tetrabromphenol blue) without altering the pH. In the absence of detectable protein, tetrabromphenol blue buffered to a pH of 3 remains yellow. Light green and then darker green to blue color develops in the presence of increasing concentration of detected protein [16, 108, 110]. The standard semiquantitative reagent test strip for protein measures total proteins in urine, but is most sensitive for the detection of albumin. Urinary albumin is usually the protein of most interest in CKD. Albumin readily binds to the indicator dye and generates the strongest color change per gram of any protein [111]. The reagent pad is less sensitive to overflow (prerenal) and tubular origin proteins that include globulins, Bence Jones protein, mucoproteins, myoglobin, hemoglobin, lysosomes, acute phase proteins, THP, and cauxin. Consequently, dipstrip protein pads better measure urinary albumin than urinary total protein or nonalbumin proteins [16, 108, 110,112–117]. Since “other” nonalbumin proteins can bind to the indicator dye at times, a positive dipstrip reaction does not always mean that this is all due to albumin [111]. The intensity of the color reaction that develops on the dipstrip pad is determined by how much protein is in the urine [107], and by the number and affinity of binding sites on a specific urinary protein [113, 115]. At least six different binding regions for albumin have been identified and three of these regions appear to be very specific [118]. It appears that the dye and protein form a specific ionic complex that depends on positive charges within the albumin molecule [119]. The degree of color development may depend in part on the dynamics of indicator dye binding to the NH3 groups on the protein. Positively charged groups on proteins are thought to increase binding to the dye [120]. The presence of positive charges and protein binding to high‐affinity sites appear to be more important than the structure of the amino acids possessing the positive charge (lysine, arginine, or histidine). Variation in dye binding to various proteins has been attributed to nonspecific electrostatic interactions [119]. Protein measurements from dipstrips are both qualitative and semiquantitative [110], if the colorimetric reaction is read on urine at room temperature at the appropriate time interval as discussed in Chapter 5. Standard urine chemistry dipstrips contain one pad that detects total protein; some dipstrips measure microalbumin. Optimal results are generated when color reactions on the dipstrip are read by an automated strip reader, which objectively reads the degree of color reaction at the prescribed time interval. An exact mg/dL of protein is not provided; the reported value represents a range (or bin) that the sample concentration is within. The degree of protein in urine is reported as 0, trace, 1+, 2+, and 3+, which corresponds to <15, 15, 30, 100, and 300 mg/dL semiquantitative values, respectively. The exact bins or cutoff values vary somewhat by manufacturer especially at the lower limits of detection [16]. Hydration status and the concentration of urine can impact protein measurement. Highly concentrated urine overestimates the degree of proteinuria/albuminuria determined on the dipstrip color reaction compared to other methods. A positive color reaction on the pad could be due to excessive protein added to the urine by some disease or it could reflect normal small amounts of protein in highly concentrated urine. False‐negative reactions for protein on the dipstrip are less common, but do occur at times in urine that is very acidic or not well concentrated (Table 7.3). The sensitivity and specificity for urine dipstrip protein reactions are low in dogs and cats [2, 8]. False‐positive reactions on dipstrip (decreased specificity) reactions to protein (compared to more quantitative bench top reference methods) are common for dipstrip reactions in both the dog and cat, but especially so for cats [2, 15]. The high occurrence of false‐positive dipstrip reactions in the cat is possibly due to the presence of cauxin. Cauxin is a carboxyesterase secreted by renal tubules into urine in high concentration in cats. Cauxin acts as an enzyme in urine to hydrolyze felinine precursors to felinine. Markedly higher urinary cauxin concentrations are encountered in intact male cats compared to neutered male, intact, or neutered female cats [127]. It appears that even low concentrations of cauxin in feline urine can activate the tetraphenol blue color indicator on the urine chemistry dipstrip. This reaction with cauxin may be the reason why so many cats without albuminuria have a dipstrip color reaction (false positive for albuminuria). Positive dipstrip reactions in this instance indicate a form of nonalbumin proteinuria that appears to be physiologically normal in cats. The selective removal of cauxin from normal feline urine resulted in negative readings during urinary dipstrip protein testing in one study [128]. Table 7.3 Interpretation of urine total protein results from urine dipstrip chemistry. Source: Lamb and Jones [16], Strasinger and Di Lorenzo [121], Sink and Weinstein [122], Sink and Weinstein [123], Rizzi et al. [124], Roura [54], Bertholf [110], Tripathi et al. [125], Miyazaki et al. [126], Miyazaki [127], Miyazaki [128], Stockham and Scott [129], Moore [130], Elliott and Sargent [131]. There is concern and confusion in the interpretation of a positive dipstrip reagent pad reaction for protein when there is also a positive reagent pad reaction for occult blood. Based on a study of dogs with hemoglobin added to urine, it required a maximal reaction on the occult blood reagent pad before the protein reagent pad turned positive from the presence of hemoglobin in the urine. This demonstrated that the dipstrip pads for protein detection were not that sensitive for the detection of protein contained in hemoglobin. In the same study, the SSA test was not sensitive for the detection of hemoglobin as it required ≥40 mg/dL of hemoglobin in order to generate a positive test [132]. Highly concentrated urine samples sometimes result in trace to 1+ protein readings that are not confirmed when measured by other methods to detect proteinuria. False‐positive reactions for protein can occur in samples with highly alkaline urine (pH >8), urine containing phenazopyridine or blood substitutes (polyvinyl compounds), and urine contaminated with benzalkonium chloride, quaternary ammonium compounds, or chlorhexidine [108, 133]. Bloody urine can obscure the interpretation of color development on the pad resulting in a false‐positive reading [116]. Dogs and cats with gross hematuria or pyuria are likely to have positive reactions for protein on dipstrip and by UPC and MA. Dipstrip reactions for protein were significantly increased in urine samples that were light pink to red in dogs and dark pink to red in cats following the addition of whole blood in one study [134]. The proteinuria or albuminuria that is reported cannot be assessed as originating from the kidneys until after gross hematuria or pyuria clears. Microscopic hematuria or pyuria is not always associated with albuminuria in the dog and cat as has frequently been considered to be true in the past. The presence of an active sediment and a large amount of urinary protein in the same sample can still be associated with renal proteinuria, but an accurate assessment is better made after analysis when the sediment is inactive [2, 93, 135]. The submission of a bacterial urine culture has been recommended to exclude a bacterial UTI that is adding postrenal proteins to urine during the evaluation for possible renal proteinuria [136]. In one study of dogs with proteinuria (UPC > 0.5), positive quantitative bacterial growth was uncommon (30/451). Sixty percent of those with positive culture had an active urinary sediment, and some combination of pyuria, bacteriuria, and a history of lower urinary tract disease predicted positive bacterial growth in most instances. A positive urine culture from dogs with proteinuria and an inactive urinary sediment was very uncommon. This finding suggests that it may not be necessary to submit a quantitative urine culture in dogs to rule out postrenal urinary infection in all instances. Submission of urine culture is still recommended when the urinary sediment is active or in those with a history of lower urinary tract disease [137]. The contamination of urine samples with semen from sexually intact dogs can increase the color reactions for protein and blood on the urine chemistry dipstrip. Sperm are sometimes found in the urine of sexually intact male dogs and cats collected by voiding, catheter, or cystocentesis. Retrograde flow of sperm into the urinary bladder occurs during ejaculation, during the use of some sedatives, and at times during routine urinary voiding [138–141]. The addition of dilutions of whole ejaculate or seminal fluid to urine of dogs increased the magnitude of dipstrip proteinuria over that from baseline. The addition of ejaculate or seminal fluid to urine also resulted in positive reactions for blood on the dipstrip in the absence of RBC in urinary sediment [142]. A negative color reaction for protein on dipstrip reliably indicates the absence of pathological proteinuria based on UPC in most instances. A negative dipstrip reaction for protein will align with a UPC < 0.5 in dogs and <0.4 in cats most of the time, regardless of USG. Dipstick protein ≥2+ are usually associated with pathologic proteinuria and albuminuria [2, 10, 11, 94]. A dipstick positive result ≥trace for protein should be confirmed with another method to measure protein irrespective of urine concentration based on USG. Trace and 1+ dipstick reactions are the most problematic to know if they indicate pathological proteinuria without confirmatory testing (UPC or MA) [11, 15]. USG and positive protein dipstrip reactions were weakly correlated in one study of dogs but USG did not add value for accurate prediction of abnormal UPC [94]. In a study of clinically normal dogs, trace (15 mg/dL) or 1+ (30 mg/dL) reactions for protein on the urine chemistry dipstrip were common. The highest UPC was 0.21 and the highest total urinary protein was 39.0 mg/dL in this population. The authors of this paper concluded that dipstrip reactions for protein at this level should be considered unremarkable instead of a falsely positive finding [143]. A substantial difference in the assigned bin for proteinuria occurred at times following visual inspection and color assessment by the technician performing the test in one study [112]. The bin for proteinuria assigned was consistent when performed by the same technician, but discordance was relatively high between technicians in human medicine. Some of the variability between protein content reported on serial urine samples can be accounted by different technicians reading the color reaction on the strip. Variation by technologist was substantial even when color blindness was excluded. Most of the discordant readings were in urine samples that were negative, trace or 1+. There was greater concordance when reading samples with stronger color reactions [112]. It is also important to remember that variation in urinary dipstrip protein readings can sometimes be attributed to different brands of reagent dipstrip used to perform the measurement [115]. Turbidimetric assays based on protein precipitation using SSA, trichloracetic acid, or heat and acetic acid are additional methods for the semiquantitative measurement of proteinuria. These methods detect albumin, globulins, and Bence Jones proteins, whereas the urinary dipstrip primarily detects mostly albumin. SSA is not sensitive for the detection of hemoglobin or myoglobin in urine. The lower limit of sensitivity of these assays is 5–10 mg/dL. Radiographic contrast agents and large doses of penicillins, cephalosporins, or sulfisoxazole as well as thymol urine preservative may result in false‐positive reactions during SSA testing. False‐negative reactions may occur with highly alkaline urine [2]. Some veterinary laboratories perform confirmatory SSA testing on urine samples that have trace or 1+ positive on dipstrip reactions for protein. Both should be positive at the same time if there is albuminuria [2]. SSA testing was abandoned by one veterinary laboratory due to its inferiority to dipstrip protein results when compared to automated microprotein assay [144]. SSA testing for urinary proteins is uncommonly performed in veterinary commercial laboratories. If the urine supernatant is not clear following centrifugation, SSA testing will overestimate the amount of urinary proteins. In one version of this test, equal amounts of supernatant and 3–5% SSA are mixed in glass tubes [2]. One part of urine is added to three parts of 3% SSA in another method. The degree of turbidity of urine supernatant that develops after the addition of SSA is subjectively estimated from 0 and trace to 4+ (Figure 7.6). 0 is perfectly clear with no turbidity (<5 mg/dL), trace has slight turbidity (5–15 mg/dL), 1+ has some turbidity but print can still be read through it (>15–30 mg/dL), 2+ is diffusely white but heavy print can still be seen (>30–100 mg/dL), 3+ is heavy white with fine precipitates and print cannot be seen (>100–300 mg/dL), and 4+ has flocculent precipitates (>300 mg/dL). A set of standard tubes with SSA reactions from 5 to 100 mg/dL increases the accuracy for the subjective scoring of the SSA reaction for proteins [2, 54, 133,145–149]. The subjectivity during visual reading the amount of turbidity that develops in urine following the addition of SSA can be removed by turbidimetric analyzers that do not depend on visual inspection [130]. FIGURE 7.6 (a)–(c) Visual inspection of urine supernatant following centrifugation, before (a) and after addition of SSA (b) and (c). The sample on the far left is negative and would be reported as 0 or <5 mg/dL; note that it is easy to read print through the sample. The sample next to the right would be trace to 1+. The sample at the far right would be 4+. Source: Used with permission of Dr. Catherine Langston, Columbus, Ohio. If the dipstrip test for protein is negative and the SSA test is positive, nonalbumin proteinuria is a consideration that includes light chains (Bence Jones proteins) and tubular proteinuria [114]. Lysozymuria is a rare type of overflow proteinuria (prerenal proteinuria) that can be detected following SSA and urinary dipstick testing [16, 150]. Increased production and urinary excretion of lysozymes can occur in some human patients with acute monocytic or myelocytic leukemia. A persistently positive urine dipstick for proteinuria in the absence of albuminuria can suggest the presence of lysozymuria, particularly in the absence of other signs of the nephrotic syndrome (NS) [150]. A positive dipstrip reading is considered falsely positive when the SSA reading is negative; the dipstrip positive readings are usually minimal or slight in these instances [107]. The measurement of UPC is useful to confirm or deny proteinuria/albuminuria initially suspected on dipstrip results, but it can also be used initially to screen for proteinuria. In the case where UPC is increased (>0.2) but the dipstrip protein reaction is minimal or zero and the MA is negative, prerenal and tubular proteinuria must be considered. UPC is a unitless number following the division of urinary protein in mg/dL by urinary creatinine in mg/dL. Urinary creatinine excretion varies little in an individual stable dog or cat over a 24‐hour period allowing for the use of this ratio. Having urinary creatinine in the denominator corrects for urine that is highly concentrated or highly diluted, as urinary creatinine concentration will be correspondingly high or low. For example: if the dipstrip is 1+ protein, urine protein on the benchtop is 70 mg/dL, and the urinary creatinine is 350 mg/dL, the UPC calculates as 0.2. If the same 70 mg/dL urinary protein were associated with a 100 mg/dL urinary creatinine, the UPC is now 0.7. Benchtop analyzers measure total protein that includes albumin and other proteins. Pyrogallol red molybdate (PRM) and Coomassie brilliant blue (CBB) are the most commonly used methods to measure urinary total proteins. Values for total urinary protein are higher when measured by PRM compared to CBB. Differences between protein concentrations measured by PRM and CBB affected International Renal Interest Society (IRIS) substaging of CKD in dogs when protein concentrations were near the cutoffs for overt proteinuria [151]. In another study, UPC was determined in proteinuric and nonproteinuric cats by either CBB or PRM methods to measure protein. Both methods were precise, but higher protein values were generated in urine measured with CBB. The coefficient of variation (CV) on replicates for CBB was higher than that for PRM. Misclassification of IRIS substaging based on UPC was similar by either CBB or PRM when values were close to the cutoff points (< 0.2 or >0.4) [152]. Aminoglycosides and gelatin volume expanders can also falsely increase the urinary total protein reported [16]. There is no universal agreement on the methods for measurement of urinary creatinine in human or veterinary medicine; all urinary methods have been modified from those initially designed to measure creatinine in serum or plasma [153]. Urinary creatinine concentrations can vary on the same urine sample when measured by different methods and analyzers. The optimal dilution for the measurement of urinary creatinine was recommended at 1:20 in general and 1:100 for samples with a USG > 1.030 in one study [154]. In a study of dog urine diluted 1:20 with distilled water, urinary creatinine concentrations were not different when measured on an autoanalyzer using the kinetic Jaffe or enzymatic methods; the degree of inaccuracy was <5% using human creatinine as a control. A trivial difference in urinary creatinine concentration determined by Jaffe and enzymatic methods is expected since interference from noncreatinine chromogens is usually very low in urine compared to that in serum. There is the potential for analytical error during the measurement of both urinary protein and urinary creatinine used to calculate the UPC, which can make the diagnostic interpretation of results less reliable. The use of benchtop analyzers has been reported to underestimate the amount of urinary creatinine for dogs (as discussed in Chapter 1). In such instances, the lower creatinine in the denominator results in an increased UPC without any change in the amount of urinary protein. This phenomenon can result in an increase of UPC such that the categorization of proteinuria is escalated from normal to borderline, and from borderline to overtly increased at times [153]. Benchtop analyzers likely to be used in‐house by veterinary practices underestimated urinary creatinine especially at concentrations >200 mg/dL; urinary creatinine concentrations were 25% lower when determined by one analyzer [153]. Consequently, it is important to base the interpretation of UPC on results obtained from reliable measurements. Urine creatinine results from benchtop analyzers may not be acceptable for clinical use, and results should not be compared to those generated from automated methods used by commercial laboratories. Both analytical and biological variability can occur during the measurement of UPC at different times on different samples from the same animal. Consequently, it is especially important to consider these factors when the reported UPC is slightly above or below the defined decision‐making points of <0.2 in the dog and cat as nonproteinuric and ≥0.4 in the cat or ≥0.5 in the dog as overtly proteinuric. The coefficients of variation for UPC in urine from dogs during repeated measurements from the same sample were 10–20% when the UPC was 0.2 (0.15–0.25) and 10% when the UPC was 0.5 (0.45–0.55) [151, 154, 155]. Consequently, a UPC slightly above or below these tipping points (UPC 0.15–0.25 for dogs and cats; 0.35–0.45 for cats and 0.45–0.55 for dogs) could result in the wrong assessment as to whether there is proteinuria or not based on this level of analytical imprecision for both urinary creatinine and urinary total protein (Figure 7.7) [154, 155]. In instances where UPC values are near defined cutoff values, the UPC should be measured at another time on another sample to determine if the UPC values are repeatable and found to be in the same category or bin of proteinuria (none, borderline, or overt) or if there is discordance. The method of urine storage can impact UPC measurement. Prolonged storage at high temperature for 30 days decreased urinary creatinine in a study of human urine, but minimal decreases were detected when stored at the same high temperature (55 °C) for two days [156]. UPC was unchanged in urine samples from dogs stored up to four hours at room temperature before measurement but UPC increased after storage at room temperature or refrigeration (4 °C) for 12 hours. There was no change in UPC when samples from dogs were immediately frozen and stored up to 3 months at −20 °C. Freezing of urine is recommended if samples cannot be immediately measured [154]. Stored samples should be analyzed after reaching room temperature and thorough mixing [16]. UPC results can also be generated from urinary dipstrips used in human medicine that contain a pad to measure urinary creatinine concentration using automated strip readers [16]. This allows UPC to be calculated and reported in semiquantitative bins. UPC screening was found to be useful in dogs but not cats in one study using a dipstrip autoanalyzer that measured urinary creatinine and protein [149]. Some discordance between UPC determined by benchtop and dipstrip analyzers is expected as they employ different methods to measure protein and creatinine. The use of urine dipstrips to accurately estimate bins for UPC needs further clinical validation in veterinary medicine. FIGURE 7.7 Imprecision in the measurement of UPC in the dog. The CV for the repeated measurement of UPC was approximately 10–20% when the UPC was 0.2 and about 10% when the UPC was 0.5. For a UPC of 0.2, the value could vary from 0.16 to 0.24. This degree of imprecision could result in assignment of the bin as either nonproteinuric or borderline proteinuric. For a UPC of 0.5, the value could vary from 0.45 to 0.55 resulting in assignment of the bin as borderline proteinuric or overtly proteinuric. It is important to consider this level of imprecision in any UPC value near the cutoff values of 0.2 or 0.5 in the dog. The UPC should be repeated to see if the same bin is assigned on subsequent samples with UPC values near the cutoffs. Similar concerns are likely for cats with cutoff values of 0.2 or 0.4 UPC. Assignment to a particular proteinuria bin should not be based on a single UPC value that is close to the cutoff, due to this degree of analytical uncertainty. UPC is generally not influenced by feeding or fasting, and whether urine is collected by cystocentesis or voiding in normal dogs and cats [8, 10]. Exposure to plastic nonabsorbent spherical pellet cat litter for one hour had no impact on feline UPC measurements [157]. In addition, UPC and urine albumin‐to‐creatinine ratio (UAC) were not different between dogs that were overweight or obese compared to those that had ideal body weight and body condition score (BCS) [158]. UPC may be influenced by the time of day that urine is collected in children [159]. In humans, there is less variation in samples collected early in the morning, as compared to samples collected at bedtime. Mean UPC measured by the CBB method in kittens from 4 to 32 weeks of age ranged from 0.14 ± 0.03 to 0.34 ± 0.18 from week to week, findings similar to that in adult cats. The UPC for cats ≥9 weeks of age correlated well to 24‐hour urinary protein excretion [160]. Mean quantitative urinary protein excretion was 52.30 ± 25.70 mg/day or 17.43 ± 9.05 mg/kg/day (4.1–42.9 mg/kg/day) in healthy adult cats as measured by the CBB method of one study, with no difference found between males and females. There was considerable variation in protein excretion in some cats on consecutive days by as much as 25 mg/kg/day [161]. Mean quantitative protein excretion in sexually intact healthy adult cats was 12.65 ± 5.45 mg/kg/24 hours and proteinuria was significantly greater in male cats (16.62 mg/kg/24 hours) compared to female cats (8.69 ± 4.09 mg/kg/24 hours). Protein in urine was measured by the CBB method. Less than 29 mg/kg/day of protein excretion is expected from healthy adult cats based on mean ± 3 SD in this study. A single UPC was highly correlated with the 24‐hour protein loss. In voided samples, the mean UPC was 0.317 ± 0.132 for all cats, 0.222 ± 0.110 in female cats, and 0.413 ± 0.065 in male cats but this did not achieve statistical significance. There was no significant difference in UPC in urine samples obtained by cystocentesis compared to voiding. The UPC of normal cats should be ≤0.7 or ≤0.65 as derived in this paper [162], which is quite a bit higher than the <0.2 currently endorsed as nonproteinuric by IRIS. Sexually intact male cats can have a higher UPC than is generally accepted as normal for neutered male and female cats. UPCs up to 0.6 were found in 124 normal young sexually intact male cats maintained in a research laboratory. All urine samples were collected by cystocentesis. The increase in UPC was likely attributed to an abundance of cauxin in the urine of intact male cats. All UPCs were <0.2 within two weeks following castration (Dr. Scott Brown, personal communication April 2020). Castration of normal dogs decreased the urinary protein detected by dipstrip and by UPC in one study (urine collected by cystocentesis) [163]. Dipstrip protein was negative in 9 and ≥1+ for 10 of 19 sexually intact normal male dogs studied prior to castration. The dipstrip for protein was negative in 13 and ≥1+ in 6 dogs after at least 15 days following castration in the same dogs. UPC was <0.5 in all the sexually intact male dogs of this study and < 0.2 following castration for all except one dog. Spermaturia was found in 15 of 19 dogs before castration and in no dogs following castration, but there was no significant association between the number of spermatozoa in the urine and the UPC prior to castration. The UPC decreased in 18 of 19 dogs following castration; mean UPC was 0.12 prior to castration and significantly decreased to a mean UPC of 0.08 following castration [163]. The magnitude of creatinine excretion into urine has not been studied in detail for dogs and cats based on age. Urinary protein excretion (mg/kg/day) and UPC increased between 4 and 14 weeks of age for normal kittens in one study [160]. Trace to 1+ dipstrip protein reactions were recorded from voided or postvoided urine samples in most puppies from an animal shelter or commercial pet stores up to 24 weeks of age [164]. In a small number of Beagle puppies, mean UPC (0.21 versus 0.10) and protein excretion (5.73 mg/kg versus 2.80 mg/kg) were higher at 9 weeks than that at 27 weeks old [165]. Total daily protein excretion was lower in puppies (6.0 ± 1.9 mg/kg) compared to mature dogs (48 ± 68.4 mg/kg) in another study [166]. It is known that the excretion of creatinine into urine declines with age in humans, which can increase the UPC despite no change in protein excretion into urine [16]. Extremes of muscle mass for any individual must be considered for its effect on the UPC. UPC may increase when urinary creatinine excretion declines with loss of muscle mass, as frequently occurs with [167]. In these instances, the UPC will increase despite the fact that urinary protein excretion has not increased at the same time. Those with a very high muscle mass and an increased urinary creatinine excretion will exhibit a decrease in the UPC [16]. The variability in UPC within a normal patient is expected to be minimal from day to day. Renal proteinuria based on UPC can be observed in apparently healthy animals. Borderline or overt proteinuria based on UPC (0.2–0.5 and >0.5 respectively) were found in 25–32% of apparently healthy senior and geriatric dogs [168, 169]. Borderline proteinuria (UPC 0.2–0.4) was encountered in 44.1% and overt proteinuria (>0.4 UPC) in 4.7% of seemingly healthy geriatric cats of one study [170]. This could indicate random biologic variability of protein filtration or an underlying disorder that has not yet been diagnosed. Variability in UPC may be more dramatic in dogs with underlying glomerular disease, as shown in one study of female dogs with stable X‐linked HN, in which UPC was measured once daily for three consecutive days. The variability in UPC was greatest in this study as the magnitude of proteinuria increased. To prove a significant difference between serial UPC, UPC had to change by at least 35% when the UPC was near 12 and by 80% when the UPC was near 0.5. One sample was considered adequate to evaluate the UPC when it was <4.0, whereas multiple samples were needed to reliably evaluate UPC with higher values [171]. Whether or not the variability in UPC demonstrated in this study also exists in other populations of dogs with glomerular disease has yet to be reported. Multiple UPCs are sometimes recommended to evaluate proteinuria in dogs with stable glomerular disease in an effort to limit the effect of random biological variability. There is inherent biological variability in the excretion of urine total protein and albumin among individual animals, so reliance on one single measurement of urinary protein can be problematic. It has been recommended to demonstrate that glomerular proteinuria is persistent and repeatable [2, 7, 8, 14, 54]. Some of this individual variability might be accounted for by genetic influences on tubular reabsorption of albumin via the cubulin receptor [16]. There was high correlation between the average of UPC from three separate urine collections and the UPC of pooled urine from samples in one study of dogs. A maximum ±20% difference in UPC from the pooled urine compared to the individual average of samples was observed in this study. Pooling of urine samples from sequential first morning urine provides a reliable cost affordable method for clients to collect voided urine specimens at home from dogs for evaluation of proteinuria [172]. UPC did not appear to differ between measurements from a single sample compared to a pooled sample in another study of dogs with proteinuria [173]. Proteinuria of renal origin is one finding that can be useful in making a decision if a patient should be staged as IRIS CKD stage 1. Patients in IRIS Stage 1 are nonazotemic and have a serum creatinine <1.6 mg/dL (C) and < 1.4 mg/dL (D) and symmetrical dimethyl arginine (SDMA) <18 μg/dL. The decision for Stage 1 is not usually made on the isolated finding of renal proteinuria, but often in some combination of findings that show reduced urinary concentrating ability, abnormal renal structure on palpation or imaging, trending increases of serum creatinine, SDMA, or renal biopsy results. IRIS CKD substaging is based on UPC and not the degree of MA [174]. IRIS recommends the use of UPC to substage CKD in dogs and cats as nonproteinuric (<0.2), borderline proteinuric (0.2 to <0.4 [cats]; 0.2 to <0.5 [dogs]), and overtly proteinuric (>0.4 [cats]; >0.5 [dogs]) (Table 7.4). Table 7.4 Proteinuria assessed by urine protein/creatinine ratio for the assignment of IRIS substage of CKD in dogs and cats. There is the possibility that the presence of RBC, WBC, and bacteria in urine may have an impact on measured protein, due to the presence of hemoglobin and plasma proteins in the urine. Urinary tract hemorrhage and inflammation are commonly considered to be causes for proteinuria [93, 183]. Clinically relevant increases in UPC developed following the addition of whole blood (urocrit 10% by volume) to urine in one study. UPC increased in urine following cystotomy and after the creation of Escherichia coli UTI in experimental dogs in the same study. The increase in UPC from lower urinary tract inflammation was not predictable based on the urinary sediment enumeration of RBC or WBC however [183]. In another study that added blood to canine urine, the UPC did not exceed 0.4 at any dilution even when urine was pink to red, and albuminuria (>1 mg/dL) did not occur until the urine was pink (RBC TNTC or >250/HPF) [93]. Median UPC values increased to >0.5 in cats and dogs when the urine samples were pink and further increased when samples were dark pink (>0.7) and red (>1.2) in another study [134]. Blood contamination led to different categorizations of proteinuria as normal, borderline, or increased based on UPC even without obvious color change to the urine in this study. A false‐positive assessment for proteinuria occurred when there were ≥250 RBC/HPF [134]. The effect of blood contamination on the UPC was more prominent in this study than in the study mentioned previously [93] prompting caution to be exercised when interpreting blood contaminated urine samples and proteinuria. Many clinical samples from dogs with pyuria did not have albuminuria or an increase in total urinary protein based on UPC in one study. There was no significant relationship between the degree of pyuria (>5 WBC/HPF) and UPC or MA [93]. The UPC was <0.5 in 41% of dogs with heavy bacterial growth in another study [94, 184]. Based on these findings, caution should be used in routinely attributing increases in albuminuria or total proteinuria to inflammation with associated pyuria or to hemorrhage (sample not red or pink). In these instances, glomerular origin proteinuria is still possible and should be reassessed following resolution of pyuria and hematuria to know with more certainty. The term “microalbuminuria” has historically been used to described increased urinary albumin excretion or concentrations in urine that are not detectable with routine urinary chemistry dipstrips. The continuing use of this term is discouraged by some in favor of the term “albuminuria” since this term refers to increased loss of albumin of any amount [16]. The measurement of MA is useful to determine loss of protein into urine below the detection of urine on dipsticks and to confirm results of positive dipstick reactions for protein [2, 12, 13]. Urine from normal dogs and cats contains <1.0 mg/dL of albumin. MA is defined as 1–29 mg/dL of urinary albumin; ≥30 mg/dL is considered overt proteinuria or albuminuria [135]. Low concentration of urinary albumin may be reported as <2 or <2.5 mg/dL depending on the laboratory and method of analysis due to lower limits of detection. Variability in MA over the same day or between days in the same patient has not been studied, as it has with UPC in some populations of dogs. Urinary albumin can be detected by both colorimetric and immunological methods [16]. Many laboratories offer urinary albumin measured by immunoturbidimetric methods designed as the gold standard for use in human urine [16, 185]. The use of species‐specific antibodies toward dog and cat albumin is not routinely employed by veterinary reference laboratories. Early studies to measure MA in dogs and cats employed a point‐of‐care analyzer using species‐specific antibodies toward albumin, but this analyzer is no longer marketed. In this method, the urine was first diluted to a USG of 1.010 to remove artifact from varying degrees of urine concentration [12, 13, 15, 145, 186]. Though the performance of this analyzer was generally acceptable as a screening test, subjectivity in the visual assessment of color development sometimes resulted in discordance with benchtop methods for MA or when compared to positive reactions on UPC or dipstrip. Reagent strips for the specific detection of human urinary albumin using colorimetry or immunoassays are available. This method can provide false‐negative results when the urine is dilute; positive albuminuria results from the dipstrip for MA should be confirmed with the standard benchtop laboratory measurement of urinary albumin and UPC [16]. One such dipstrip performed poorly when protein in urine from dogs was assessed using a dipstrip reader [187]. MA was measured by VetScan UA 14 strips with an automated analyzer that employs fluorescein dye as a nonspecific binder for proteins. MA is reported as either <2.5 mg/dL (negative) or ≥2.5 mg/dL (positive). All quality control materials that were negative or positive generated results that were in the correct bin for MA, but demonstrated a negative bias at lower albumin concentrations. Immunoturbidometric or ELISA testing that generates a numerical value was recommended instead of relying on these two bins of MA assignment. MA measured at a referral laboratory is needed to determine a specific value for MA between 2.5 and 29 mg/dL and to determine a baseline to document trends. It is more appropriate to follow UPC when MA values exceed 29 mg/dL [135]. MA positive status was found in 25% of apparently healthy dogs [188] and 9–14% of apparently healthy cats [15, 186]; this increases with age and when a dog or cat is ill. MA positive status was found in 31% of dogs and 26% of cats with various clinical conditions [12, 13]. MA occurred in greater than 50% of critically ill dogs, a finding that was more frequent than an increased UPC [189]. MA positive status develops in dogs and cats early on in many disease processes, often before UPC is overtly increased. The same diseases shown to be associated with increased UPC usually have MA positive status at the same time. It appears likely that MA becomes positive first, followed by UPC, and then dipstrip positive for protein in diseases with progressive glomerular injury [135]. Based on the UAC, MA (UAC ≤ 0.3) was detected in 32.5% of dogs with CKD and macroalbuminuria (UAC > 0.3) in 50% of dogs with CKD [190]. In general, a UPC > 1.0 was associated with macroalbuminuria, UPC of 0.5–1.0 was associated with MA, and a UPC < 0.5 was not associated with albuminuria in this study [190]. MA occurred before overt proteinuria was detected by UPC in dogs with X‐linked nephritis in both male [191] and female dogs [192]. Similarly, the prevalence of MA was high in soft‐coated Wheaton terriers (SCWTs) genetically predisposed to develop glomerular disease and MA increased with age. MA occurred more frequently than a UPC > 0.5 in this population [193]. The detection of MA can indicate the presence of early glomerular damage not detectable by other methods [11]. Albuminuria that is persistent or of increasing magnitude may be the earliest clinical indicator of glomerular disease [93]. Systemic inflammation can be associated with the endothelial leakage of protein and MA. Dogs with severe inflammatory response syndrome (SIRS) frequently have increased UPC and MA due to glomerular barrier dysfunction [194]. Deposition of immune complexes within the glomerulus, as well as decreased blood flow to the kidneys and injurious molecules synthesized by tumors, is thought to contribute to glomerular injury and proteinuria in patients with neoplasia [195]. The long‐term effects or associations of stable MA or borderline UPC levels on renal function or survival during primary glomerular disease or systemic disease are not known [15]. Clearly, not all MA positive animals develop CKD, as there are many more MA positive status dogs and cats than are diagnosed with CKD. Nearly 25% of over 3000 dogs owned by veterinary hospital personnel were found to have MA positive status in one study. MA positive status increased in frequency with age from 4% at 1 year to 55% at 15 years of age. Of the 572 dogs with MA positive status that were followed, 56.3% were diagnosed with underlying infectious, inflammatory, neoplastic, or metabolic disease. Dogs with renal disease accounted for 31%, 12.1% had no specific diagnosis, and 0.6% had multiple diagnoses or ones not related to proteinuria [12, 188]. Forty‐five percent of apparently healthy dogs were found to be MA positive using a dipstrip automated reader to measure albumin and creatinine in another study [196]. In another study of dogs examined at a teaching hospital that were negative for protein on dipstrip chemistry, the association between MA status and diagnosis within three months was determined. MA positive status was shown in 111 of 352 (31.5%) dogs with one diagnosis. Of dogs with positive quantitative MA status, 6% were diagnosed as healthy, 31% with neoplasia, 20% with infectious‐inflammatory‐immune‐mediated diseases, 14% with urinary disorders, and 29% had diseases that did not fall into any of the other categories. Quantitative MA had a sensitivity of 35.6% and specificity of 85.4% for the detection of systemic disease in dogs [12]. MA was documented in 14% of 611 apparently healthy cats, and its frequency increased with age [15]. At a teaching hospital, MA positive status was shown in 85 of 324 (26%) cats. Of cats with MA positive status, 2% were diagnosed as healthy, 11% with neoplasia, 20% with infectious‐inflammatory‐immune‐mediated diseases, 33% with urinary tract disease, 16% with endocrine disease, and 18% with other diseases that did not fall into any of the other categories. Quantitative MA had a sensitivity of 37% and a specificity of 94% for the detection of systemic disease in these cats [13]. MA by semiquantitative strip testing was found in 36% of sick cats and 9% of healthy cats in another study. MA was positive in more samples than for increased UPC as expected, but there were instances in which UPC was increased but MA was negative. Either the MA was falsely negative or proteins other than albumin accounted for the increased UPC [186]. MA status was evaluated in 599 canine and 347 feline urine samples from apparently healthy animals [145]. The ability of positive dipstrip protein reactions to predict MA was high in the dog and very high in the cat, but false positives on dipstrip for protein were frequent, especially in the cat. The finding of positive results for both SSA and dipstick for protein decreased the number of false‐positive results but did increase the number of false negatives in both species compared to MA. When ≥2+ protein reactions were considered as the cutoff for positive protein on the dipstrip, very few false positives were diagnosed, but false negatives based on MA increased [145]. A total of 239 urine samples from 37 cats with CKD were evaluated for proteinuria. Positive results from dipstick and SSA together, SSA alone, or dipstick ≥2+ were indicative of albuminuria. The higher the dipstick reaction for protein, the lower the number of false positives. Conversely, lower dipstick reactions for protein increased the number of false negatives. The performance of dipstick and SSA positive reactions in combination was better in CKD cats than in apparently healthy cats for the identification of MA positive status. Highly concentrated urine can result in false‐positive reactions for protein on the dipstick that are not associated with MA positive status, a situation which is more likely to occur in healthy cats as CKD cats often have much less concentrated urine. A UPC of ≥0.2 as a cutoff performed well for the identification of MA in CKD cats of this study [148]. The concentration of total urinary protein or urinary albumin is measured clinically, but specific proteins can be quantitated by immunoassay or specialized electrophoresis at times. Urinary proteins can be separated according to mass and electrical charge using high‐resolution gel electrophoresis techniques (SDS‐PAGE). This method allows urinary proteins to be separated into origin as glomerular, tubular, or mixed pattern [16]. As glomerular damage increases, the permeability of the glomerulus to plasma proteins increases. This allows intermediate‐molecular‐weight proteins, such as albumin (67 kD), to appear in the urine first followed by the appearance of higher weight proteins (HMW ≥ 100 kD, such as immunoglobulins) as more glomerular damage occurs [6]. Urinary protein electrophoresis was helpful in classifying renal proteinuria as glomerular or tubular in origin in dogs of one study. Glomerular origin protein was likely when >41.4% of the total proteins in urine consisted of albumin and when the ratio of urinary albumin to alpha‐1 globulin was >1.46 [197]. Urine protein banding patterns were correlated with the degree of histopathological glomerular or tubulointerstitial injury in another study [5]. LMW proteinuria at <69 kD typifies patients with tubular proteinuria. LMW proteins are freely filtered across the glomerulus and would normally be reabsorbed by healthy proximal tubules, so the detection of these proteins reflects the failure of tubular reabsorption [3, 5, 16, 135, 198]. When excess albumin enters tubular fluid from glomerular disease, LMW proteinuria can also occur following the saturation of proximal tubular mechanisms for the absorption of proteins, resulting in both albuminuria and the appearance of LMW proteins in urine. Additionally, persistent high‐level exposure to protein in proximal tubular fluid can induce toxic lesions to proximal tubular cells, which further impairs their ability to reabsorb protein [6, 199]. Tubular proteinuria is expected in some forms of AKI and CKD from impaired proximal tubular reabsorptive function independent of tubular fluid protein toxicity. Progressive glomerular damage often results in some combination of renal proteinuria, reduced GFR, and glomerular lesions that can be identified on renal histopathology (microscopy by light, EM, and IFA), but the finding of renal proteinuria (urine reagent strip, UPC, or MA) is the most sensitive indicator of early renal disease, in which glomerular permeability to primarily albumin has increased [200]. The magnitude of glomerular proteinuria is considered a marker for the severity of glomerulopathy (Table 7.5) [20, 201, 202]. Though not conclusively proven in dogs and cats with CKD, it is likely that renal proteinuria serves as a marker of underlying glomerular disease as well as a contributor to the progression of further renal injury. Similarly, the magnitude of proteinuria indicates the degree of glomerular involvement rather the diagnosis of a specific glomerular disease [203–205]. A UPC < 0.2 is normal for most dogs and cats. Borderline values are from 0.2 to <0.4 for the cat and from 0.2 to <0.5 for the dog. Values ≥0.4 for the cat and ≥0.5 for the dog are considered overtly proteinuric according to IRIS guidelines. Proteinuria based on the UPC generally increases with an increase in CKD stage. It is often accepted that a UPC > 2.0 in CKD is associated with primary glomerular disease and HMW proteinuria that includes albuminuria [2, 14, 136]. The notion that dogs with UPC > 2.0 usually have glomerular proteinuria does not always hold true. In dogs with biopsy proven CKD, those with tubulointerstitial disease had a median UPC of 3.9 (0.6–8.6). Some of the increase in UPC in these instances was attributed to secondary damage to glomeruli [60]. The finding of a low UAC or low MA could be helpful in supporting tubular proteinuria in those with a high UPC. Similarly, the analysis of SDS‐PAGE can be used to confirm a preponderance of lower molecular weight proteins that occur with tubular origin proteinuria. Tubular proteinuria alone should not result in decreased circulating albumin as can occur with high‐magnitude albuminuria. Table 7.5 Hypothetical interpretation of various combinations of proteinuria results by dipstrip, SSA, UPC, and MA. UPC – urinary protein to creatinine ratio; d – dogs; c – cat. a MA – microalbuminuria; negative <2.5 mg/dL; MA positive ≥2.5 mg/dL. In another study, dogs with IRIS CKD stages 3 and 4 had a predominant pattern of tubular proteinuria based on electrophoretic pattern. In the same study, some dogs with CKD IRIS stage 4 and a UPC > 2.0 had predominantly LMW proteinuria characteristic of tubular proteinuria, indicating that current UPC guideline suggestions can result in a flawed interpretation for the origin of the proteinuria at times [3]. THP significantly decreased as the severity of CKD increased based on the IRIS CKD stage suggesting that THP could be used as an indicator for the progression of CKD in dogs [3]. Cats with advancing CKD have less proteinuria than in dogs with CKD since cats appear to have less glomerular disease and more tubulointerstitial disease. Despite less proteinuria for cats compared to dogs with CKD, survival was significantly related to the degree of proteinuria based on UPC in two studies. Cats with UPC < 0.2 lived the longest, and cats with >1.0 or >0.4 UPC lived the shortest times [167, 206]. Dogs with UPC ≥ 1.0 at the time of CKD diagnosis did not live as long as dogs with UPC < 1.0 and were at greater risk for a uremic crisis [207]. It is not clear if acute stress can increase the UPC in veterinary patients, though this notion has been considered possible [2, 10]. Increases in circulating cortisol are one aspect of the stress response that could have an impact on the UPC. Chronic increases in circulating cortisol from hyperadrenocorticism (HAC) or from exogenous administration of glucocorticosteroids can increase the UPC in dogs [208]. Healthy dogs were more stressed when in the hospital compared to when at home as assessed by the urinary cortisol‐to‐creatinine ratio and results of a client questionnaire, but stress had no impact on the UPC in this study. The UPC determined in voided samples from healthy dogs with low level UPC did not differ between samples collected in the hospital (median 0.03) or at home (median 0.02), and there was no relationship between time spent in the hospital, travel time to the hospital, or elapsed time until sample collection on UPC [209]. In dogs with a UPC > 0.5 in another study, the collection of voided urine in the hospital was associated with an increase of UPC compared to samples collected at home, but an index of stress was not evaluated. There was no impact of the urine collection site when the UPC was <0.5. The magnitude of increase in the UPC in some of the in‐hospital collected samples was substantial [210] and would affect IRIS CKD substaging based on the UPC. In dogs with PLN receiving at least one month of treatment with ACE‐I, angiotensin II receptor blocker (ARB), or both, the effect of urine collection at home or in the hospital on the magnitude of UPC was reported. The UPC was higher when urine samples were collected in the hospital compared to urine samples collected by owners at home, both when the UPC was >4.0 and ≤4.0. The determination of a UPC from a single sample, the average of multiple samples, or from a pooled sample was considered comparable in this study [173]. Serial UPC is helpful to monitor the efficacy of dietary or drug therapy to mitigate proteinuria during CKD. The magnitude of proteinuria and trends for increasing or decreasing proteinuria over time are important considerations in determining how aggressive the diagnostic approach and treatment should be (Figure 7.8a, b). In nonazotemic dogs and cats, proteinuria should be monitored when MA is positive and/or UPC ≥ 0.5 to <1.0, investigated when the UPC is between 1 and 2, and intervention should occur when the UPC is 2 or above. In azotemic dogs and cats, proteinuria should be monitored and investigated when MA is positive and/or the UPC is between 0.2 and 0.4 in the cat, and 0.2 and 0.5 in the dog. Intervention should occur when the UPC is 0.4 or above in the cat and 0.5 or above in the dog [14]. Progressive increases in albuminuria characterize many active glomerular diseases. It should be noted that severe reductions in GFR from loss of nephron mass potentially can limit the degree of expected rise in proteinuria due to loss of glomerular surface area available for the filtration of plasma proteins as nephron mass is lost [167]. In these instances, a reduction in UPC is not associated with improvement in glomerular barrier function, but this phenomenon is uncommon. Persistence of protein positive status based on UPC or MA ideally should be confirmed three times two or more weeks apart (Figure 7.9a) [7, 14]. If MA is positive and UPC is overtly positive, UPC (and not MA) is monitored in the future. If MA is positive and UPC is normal, MA should be followed to see if the magnitude of MA is increasing over time. A low level of MA that is not increasing may reflect previous damage or a disease process that is no longer active. A low level of MA that increases over time is a cause for concern that a primary or secondary disease process may be progressively damaging the kidneys. In a patient with MA positive status on qualitative dipstrip screening, it can be advantageous to send an MA to a reference laboratory to get a specific value (1–29 mg/dL) rather than just knowing it is ≥2.5 mg/dL from the dipstrip. The finding of MA during routine clinical visits offers the clinician a chance to investigate and diagnose systemic and primary renal disease processes before the patient is clinically ill and before UPC is increased (Figures 7.9a, b). The risk for persistent MA positive status to result in progressive CKD is not known. More effort is directed to the investigation and treatment of renal proteinuria that is persistent, of high magnitude, and that is sequentially increasing in magnitude even when not yet above the reference range (Figure 7.9b) [7, 10, 14]. It has been suggested that dogs with higher level MA > 20 mg/dL and those that have increasing levels of MA over time are at the greatest risk to develop further glomerular injury [219]. When renal proteinuria persists or escalates from lower levels, the patient should be evaluated for CKD, and systemic processes ruled out. Once CKD is suspected as the cause for the proteinuria, a renal biopsy could be performed for a definitive diagnosis. Following the diagnosis of CKD, an IRIS stage should be assigned, and treatment for renal disease, systemic disease, renal proteinuria, and hypertension initiated. FIGURE 7.8 (a) Recommended scheme of responses for clinicians based on the magnitude of proteinuria in nonazotemic dogs and cats (2005 ACVIM Consensus Statement) [14]. The intensity of monitoring proteinuria, investigating the cause of renal proteinuria, and treatment of renal proteinuria increases as the magnitude of proteinuria increases. Note that no decisions are based on proteinuria determined by urine chemistry dipstrip. The effect of renal proteinuria on survival in nonazotemic animals is not known in dogs, but has been reported to have a negative impact on survival in cats [211]. The documentation of renal proteinuria in nonazotemic dogs and cats can be used as one factor in deciding if IRIS CKD stage 1 should be assigned. There is no evidence that intervention at lower levels of proteinuria change outcome. MA – microalbuminuria; UPC – urinary protein to urinary creatinine ratio. Source: Figure redrawn from Lees et al. [14]. Illustration by Tim Vojt, reproduced with permission of The Ohio State University. (b) Recommended responses for clinicians based on the magnitude of proteinuria in azotemic dogs and cats (2005 ACVIM Consensus Statement) [14]. Note that the threshold for responses is based on lower levels of proteinuria when the dog or cat is azotemic than when nonazotemic. MA – microalbuminuria; UPC – urinary protein to urinary creatinine ratio. Source: Figure redrawn from Lees et al. [14] by Tim Vojt © College of Veterinary Medicine – The Ohio State University. Illustration by Tim Vojt, reproduced with permission of The Ohio State University. Familial nephropathy is not a single disease, but the use of this term provides an umbrella to include renal dysplasia, renal cortical hypoplasia, juvenile renal disease, glomerulopathy, HN, some forms of renal amyloidosis, polycystic kidney disease, and tubular transport dysfunctions [30, 220–222]. Familial glomerulopathy is used to include all conditions with a predominantly glomerular component. Familial glomerulopathy has been described in over 24 dog breeds, and the genetics involved in dogs and cats has been extensively reviewed [30, 221, 223, 224]. Early onset renal origin proteinuria characterizes these diseases. Many of these diseases are discovered during the documentation of proteinuria and progressive CKD in related young patients, but in some the diagnosis is made in older animals. Genetic mutations can alter the molecular composition of the GBM and the podocyte slit pore membranes leading to proteinuria and progressive CKD, the severity of which will vary based on the specific genetic defect. Much remains to be discovered about the molecular and biochemical alterations within the glomerulus that result in proteinuria and progression of CKD and the specific associated genetic defects. HN occurs in a few dog breeds. HN is the term used to describe a rare form of progressive CKD due to a genetic defect in type 4 collagen of the GBM. Progressive renal proteinuria occurs as the first clinical abnormality during the development of HN. Multilaminar splitting and thickening of the GBM on EM and abnormal immunolabeling of alpha chains from type 4 collagen characterize HN. Mutations in gene translation result in abnormalities that prevent the assembly of the full complex of side chains that are needed for normal GBM structure and function [221, 225]. Samoyed [226] and mixed‐breed dogs in Navasota, Texas [221, 227] have an X‐linked form of HN, whereas English Cocker Spaniels [228] and English Springer Spaniels [229] have an autosomal recessive form of HN. Canine HN is a rare disease that should be considered whenever a dog exhibits a juvenile‐onset kidney disease characterized partly by proteinuria [221]. Early reports of Cocker Spaniels with apparent familial nephropathy and proteinuria were described to have renal cortical atrophy. At that time, it was not determined if the cortical atrophy and reduced number of nephrons were from hypoplasia at birth or an acquired loss of renal tissue later [230–233]. It was later discovered that this syndrome was not from a congenital lack of renal cortical tissue, but that it was associated with a primary glomerular disease and progressive proteinuria [234, 235]. Primary renal glucosuria was also occasionally documented in this syndrome [232]. The incidence of HN in English Cockers has dramatically declined since the development of a genetic test that identifies carriers in this breed [221]. A specific polymerase chain reaction (PCR) test is available to identify English cocker spaniel carriers at www.pawprintgenetics.com [30]. There is an X‐linked dominant HN in male Samoyed dogs characterized by renal proteinuria and renal failure. The characteristic ultrastructural lesion is multilaminar splitting of the lamina densa of the GBM [236, 237]. This disease is caused by a single gene mutation that leads to a severe reduction in alpha‐5 chains of collagen type 4 in the GBM [226]. Defective cross linking of collagen type 4 appears to lead to weakening of the GBM [238]. A PCR test for this mutation is available and can be ordered at www.vetgen.com; www.pawprintgenetics.com. The use of genetic testing results allows the removal of carrier dogs from a breeding program [30]. FIGURE 7.9 (a) Algorithm for a general approach to renal proteinuria. Qualitative MA has historically been measured by point‐of‐care devices (Heska® ERD) that are no longer in the veterinary market. The qualitative measurement of MA (negative or positive) for dogs and cats is under current exploration by some veterinary laboratories and may be feasible as a screening test on expanded urinary chemistry dipstrips. The accuracy of this measurement compared to benchtop quantitative measurement has not been established. Alternatively, this algorithm can be started when MA is determined quantitatively. Source: Lees et al. [14], IRIS [174], Whittemore et al. [13], Whittemore et al. [12], Harley and Langston [11]. Note: RBC – Red blood cells, WBC – white blood cells, HPF – high powered microscopic field, MA – microalbuminuria (dipstrip and bench methods), UPC – urinary protein to urinary creatinine ratio, CKD – chronic kidney disease, AKI – acute kidney injury. (b) Algorithm showing an approach to determine the diagnosis for renal proteinuria. * Renal biopsy is not advocated for all patients with CKD, especially if the kidneys are small and there is advanced disease. Renal biopsy is helpful to disclose immunological and nonimmunological causes for those with renal proteinuria before there is obvious azotemia and extensive renal fibrosis. Pathological findings from the combination of light microscopy using special stains, immunofluorescent microscopy, and electron microscopy are needed in order to properly assess underlying renal pathology causing renal proteinuria. Glomerular causes of renal proteinuria are most common. The prognosis for patients with renal proteinuria varies by the specific pathological diagnosis. Aggressive treatment protocols using immunosuppressive drugs are best directed in patients that have undergone renal biopsy providing evidence for an immune process (e.g. immune‐complex deposition within the glomeruli). Source: Cianciolo et al. [27]; Segev et al. [212]; Brown et al. [136]; Schneider et al. [60]; Littman et al. [213]; Goldstein et al. [214]; Pressler et al. [215]; Cowgill and Polzin [216]; Cianciolo et al. [61]; Klosterman et al. [217]; Lees et al. [14]; Dambach et al. [218],. The International Veterinary Renal Pathology Service (IVRPS) processes renal tissues and examines them with the special techniques mentioned above, and then a group of veterinary nephropathologists consult to deliver a final diagnosis. Further information about how to handle and submit renal tissue and fees can be accessed on line form the IVRPS at https://vet.osu.edu/vmc/international‐veterinary‐renal‐pathology‐service‐ivrps. Note: AKI – acute kidney injury, BUN – blood urea nitrogen, SDMA – symmetrical dimethyl arginine, USG – urine specific gravity, FeLV – feline leukemia virus, FIV – feline immunovirus, CKD – chronic kidney disease, IRIS – International Renal Interest Society, Light – light microscopy, IFA – Immunofluorescent antibody, EM – electron microscopy, MA – microalbuminuria, UPC – urinary protein to urinary creatinine ratio. Detailed reviews of the structure and function of podocytes as well as their response to injury and development of proteinuria are available for the interested reader [23, 36]. Stretching of podocytes is now recognized as a central mechanism contributing to podocyte injury, proteinuria, and progressive CKD. Stretching and shear forces within the glomerulus contribute to foot process simplification, foot process effacement, detachment of podocytes, and loss of podocytes, all of which contribute to the development of proteinuria [23, 36]. The earliest podocyte lesions include foot process simplification and effacement; later lesions are associated with death and loss of podocytes. Live and dead podocytes can be identified at times in the urine in these instances. Typical kidney biopsy lesions in humans include minimal change glomerular disease and focal segmental glomerulosclerosis (FSGS) [36]. Several types of mechanical shear forces are thought to contribute to podocyte injury and fragility. Initial hydrostatic forces during glomerular filtration create circumferential stress on podocyte foot processes, followed by shear stress on the lateral aspects of foot processes, and then lateral flow force across the podocyte cell body. Higher hydraulic pressures develop in single nephrons as part of the adaptive/maladaptive response to loss of nephron mass (intraglomerular hypertension and hyperfiltration) in those with progressive CKD, which increases shearing forces. Loss of podocytes reduces the density of the podocyte population within glomeruli which increases shearing forces transmitted to the remaining podocytes. Also, an increased amount of protein in Bowman’s space in those with proteinuria increases the oncotic pressure and fluid drag that favors a higher single‐nephron GFR, adding to the magnitude of glomerular hypertension and shearing force [36]. Loss or altered function of nephrin and podocin molecules within the slit pore membrane and loss of podocytes (podocytopenia) contribute to proteinuria in some glomerulopathies affecting humans [31, 36, 99, 101]. The slit pore membrane can also be damaged by immunological mechanisms in addition to that caused by genetic defects. Genetic podocytopathy has recently been identified in dogs. Following podocyte loss and exposure of denuded GBM, surviving podocytes undergo hypertrophy in an attempt to cover the GBM. The development of hypertrophic podocytes, however, is considered a maladaptive response at times when foot process structure and function do not develop, allowing increased shearing forces to be transmitted. Parietal podocytes in Bowman’s capsule can deliver some podocyte progenitors, potentially contributing to recovery of podocyte function within the glomerulus, but this process can be inefficient and maladaptive with scar formation [36]. An adult‐onset PLN is estimated to involve 5–15% of SCWT dogs. More females are affected than males, but this is not sex‐linked genetically [219, 239, 240]. The mode of inheritance is considered complex, as both recessive and dominant inheritance patterns can be observed and environmental triggers for gene expression may be important. PLN is the result of an inherited podocytopathy in some SCWT dogs that involves mutations of a single nucleotide in the genes NPHS1 and KIRREL2. These genes control the synthesis of nephrin and Nephr3/filtrin that are important proteins required for normal structure and function of the podocyte slit pore membrane. Dogs that are homozygous for both mutations are more seriously affected [239]. Alterations in these slit pore proteins reduce the integrity of the glomerular barrier with resulting proteinuria. FSGS along with podocyte degeneration and loss are the most consistent renal lesions on light microscopy due to genetic podocytopathy and slit diaphragm protein defects. These defective podocytes are considered at increased risk for injury by other mechanisms (inflammation or circulating immune complexes) [30, 219]. PLN most often is diagnosed alone, but it can be preceded by protein‐losing enteropathy (PLE), or diagnosed concurrently with PLE in this breed. SCWT with both PLE and PLN have a higher degree of proteinuria based on UPC compared to PLN alone, suggesting that proteinuria can be aggravated by other insults in carriers [30]. Some affected dogs with MA at low levels can be observed without progression to overt renal disease, whereas other dogs with higher levels of MA show more rapid progression of CKD [219]. Early studies of PLN in this breed reported dogs with advanced CKD, hypoalbuminemia, and a poor prognosis [240]. Very early reports of familial nephropathy in this breed did not have proteinuria, and it appears that the likely diagnosis was renal dysplasia [241]. Genetic testing for variant genes affecting podocytes can be ordered at http://www.scwtca.org/health/dnatest.htm [30]. Of 214 breeds screened, only Airedale Terriers were found to have the same genetic defect associated with podocytopathy and risk for the development of CKD as described for SCWT. There are many opportunities for genetically mediated podocytopathy to develop not involving the specific genes shown to affect SCWT and Airedale Terriers [30, 224, 242]. FSGS was described at necropsy in eight related Miniature Schnauzers with persistent renal proteinuria (UPC 1.2–6.5) and minimal to absent azotemia. Proteinuria was usually adult onset and hypoalbuminemia rare. The progression of CKD was slow and systemic hypertension mild to moderate. These dogs were part of a long‐term study investigating renal disease in this breed. Glomerular synechia and hyalinosis were commonly identified by light microscopy. Extensive effacement of podocyte foot processes was characteristic. There was no splitting of the GBM or identification of electron dense deposits in the GBM. Glomeruli contained varying amounts of lipid in sclerotic areas and unusual electron dense aggregates were prominent within podocytes. A genetic podocytopathy with resulting podocytopenia was suggested to cause FSGS in these dogs. An underlying podocytopathy could render the glomeruli “fragile” and predispose to further renal injury from hyperlipidemia, glomerular hypertension, or other unidentified stressors. Renal‐related death did not occur in this population [243]. Schnauzers with proteinuria accounted for 3% of all renal biopsies that were submitted from dogs with proteinuria for comprehensive evaluation in another study; FSGS was the diagnosis in 78% of these biopsies [60]. FSGS has been reported in 20.6–33% of dogs undergoing comprehensive renal biopsy for renal proteinuria, second in frequency to immune‐complex‐mediated glomerulopathy [60, 74]. A similar incidence for the diagnosis of FSGS was reported in 26% (77 of 299) of dogs in another study detailing clinical pathology and prognosis. Significantly more females were affected than male dogs. Systemic hypertension was common, but azotemia and severe hypoalbuminemia were uncommon. The median UPC of 5.9 (1.4–22) was not associated with survival, but the degree of renal proteinuria and systemic hypertension control during treatment could not be determined in this study. Decreased survival was related to serum creatinine >2.1 mg/dL and serum albumin <2.0 g/dL. The magnitude of proteinuria based on UPC did not differentiate immune‐complex from nonimmune‐complex glomerular disease [244]. Mechanisms of renal damage leading to glomerular proteinuria include deposition of immune complexes, complement activation, cytokine activation, and precipitation of complexes within glomeruli [245]. When complement activation is unrestricted, it overcomes the protection of complement regulators, leading to cellular injury [246]. Immune‐complex deposition from the circulation is likely the most common process within the glomerulus. It appears that a delicate balance between antigen and antibody concentrations is required in order to form the antigen–antibody complexes likely to be deposited in the glomerulus. A mild excess of circulating antigens favors the formation of antigen–antibody complexes [247]. Rarely, autoantibodies can react with normal antigens within the glomerulus, which appear as a smooth linear pattern on immunofluorescent microscopy. Alternatively, antibodies from the circulation can combine with antigens previously planted within the basement membrane (Figures 7.10a, b), resulting in “lumpy‐bumpy” immune complexes on immunofluorescent microscopy in both examples. Rarely, autoantibodies can react with normal antigens within the glomerulus, which appear as a smooth linear pattern on immunofluorescent microscopy. Cytokines and oxygen radicals are generated along with an influx of inflammatory cells in reaction to these immune complexes. Proteinuria results as these processes disturb the delicate relationship between the various components of the glomerulus that are needed to maintain glomerular barrier integrity [99]. The size and solubility of circulating immune complexes impact where they can be deposited within the glomerulus. Small soluble complexes tend to be deposited just below the visceral epithelium (subepithelial) within the GBM though they can be deposited deeper within the GBM also (intramembranous). Intermediate size complexes are more likely to be deposited within the mesangium, whereas large immune complexes never make it to the kidney since they are removed by phagocytosis [248, 249]. Definitive diagnosis for ICGN requires the evaluation of microscopy by transmission EM and immunofluorescence (Figure 7.11) [27, 60]. Even when it is known that ICGN is the underlying renal lesion, antigens within the immune complex are rarely identified [213, 250]. Idiopathic membranous nephropathy was reported in 11 cats and 5 dogs that underwent the extensive evaluation of renal tissue by light microscopy, immunofluorescence, transmission EM, and scanning EM. Most of these animals were presented in the NS, and some had azotemic CKD. Proteinuria was heavy with most animals having >1000 mg/dL. Immunofluorescence for IgG and C3 as well as electron dense glomerular deposits was demonstrated in all the renal tissues studied, but the antigen(s) were not identified [251]. FIGURE 7.10 (a) Immune‐complex glomerulonephritis. Circulating immune complexes become trapped as subendothelial deposits, within the basement membrane, or subepithelial deposits. The location of the deposits depends on the size of the complex and the nature of the antigen. Chronic mild antigenic excess is considered necessary in order to stimulate the right ratio of antibody to antigen for this to occur (infectious agents, neoplasia). The location of the complex within the glomerulus can determine how inflammatory a reaction will develop. Source: Illustration by Tim Vojt, reproduced with permission of The Ohio State University. (b) In this scheme, antigens were planted within the basement membrane from the circulation first and then circulating antibodies bound to the antigen in situ. Alternatively, the antigen within the basement membrane is normally there and antibodies develop to this antigen as part of an autoimmune disease, though this is rare in dogs and cats. Source: Illustration by Tim Vojt, reproduced with permission of The Ohio State University. FIGURE 7.11 Immune‐complex glomerulonephritis (ICGN). IFA renal biopsy 40× –pathology consensus report from the International Veterinary Renal Pathology Service (IVRPS). Global diffuse granular mesangial staining against C3 (lumpy bumpy, granular discontinuous pattern). Not shown: Equivocal fine granular staining for IgG. Diffuse global mesangial staining for IgM. Negative staining for IgA. Based on the finding of IFA positive results and electron dense deposits on TEM (not shown), the diagnosis of immune‐complex glomerulonephritis was concluded. The nature of the antigen is not known, but there were some features on EM (not shown) that suggested a condition similar to lupus nephropathy in humans. An ANA titer or LE prep were never analyzed. Over the next 15 months, the dog was treated with enalapril and spironolactone for proteinuria control, amlodipine to achieve normal blood pressure, and anti‐platelet treatment with Plavix® to help prevent thromboembolism. She initially received immunosuppressive therapy with prednisone tapered over two months, and then was maintained on chlorambucil. Over that last year, she gained 3–5 kg from her lowest weight and the degree of her azotemia declined (serum creatinine of 1.9 mg/dL). She is still proteinuric but to a lower magnitude (UPC of 3.6) and her hypoalbuminemia abated (serum albumin of 3.3 g/dL). Her hypertension is well controlled (systolic blood pressure of 140 mmHg), which helps to lessen proteinuria in addition to the immunosuppressive treatments. An underlying genetic podocytopathy, disorder of GBM, or immunodysregulatory defect may trigger abnormal immune‐complex handling within the kidneys [30]. This could predispose animals to infection‐associated GN, as immune complexes could more readily accumulate at these abnormal sites in the glomerulus. Animals with an underlying genetic podocytopathy conceivably could have a very sensitive or fragile podocyte population that when exposed to an accumulation of immune complexes would result in greater proteinuria and progression of CKD. It has been speculated that the alteration of the molecular structure or the quantity of proteins within the slit pore diaphragm could cause abnormal folding of that protein, leading to subtle or overt proteinuria depending on the magnitude of the defect (Littman, personal communication 2019; Cianciello, personal communication 2019). can result in a varying magnitude and type of renal proteinuria, though CKD and chronic glomerular injury most commonly cause renal proteinuria. Tubular origin proteinuria can develop during AKI secondary to nephrotoxins, renal ischemia, or leptospirosis. The magnitude of this proteinuria is usually considered as mild to moderate (0.5–2.0 on UPC) and occurs secondary to proximal tubular injury. UPC > 0.5 occurred in 77% of dogs with AKI in one series with a variety of underlying causes and 58% had a UPC > 2.0. The median UPC in these dogs was 1.68 (0.1–18). Fluid overload and systemic hypertension were common in this population [252] and likely accounted for some of the renal proteinuria. Predominantly glomerular involvement in AKI is not common, so a very high degree of albuminuria is not expected to increase the UPC in typical AKI in which tubular damage is expected to predominate. AKI can decrease the proximal tubular reabsorption of the small quantities of albumin as well as LMW proteins in tubular fluid. Tubular proteinuria is expected to increase during nephrotoxic, ischemic, and some infectious causes of AKI that could account for an increased UPC in some instances, in addition to glomerular injury. In a study of dogs with different causes of AKI, the median UPC in survivors was 1.4 (0.1–14.7) and 2.7 (0.2–72) in nonsurvivors was significantly different. The median UPC was 1.62 (0.09–72.00) for all dogs with AKI, 2.21 (0.09–72.00) for those with intrinsic AKI, 1.00 (0.10–10.15) in those with volume response AKI, and 0.07 (0.04–0.28) in controls. Further characterization for the nature of the proteinuria was not reported [253]. The median UPC was 2.14 (0.40–184) in dogs with AKI due to leptospirosis compared to 2.38 (0.09–72) in those with other causes of AKI and 0–0.5 in controls. The nature of the proteinuria was not characterized further [254]. The median UPC in 64 dogs with leptospirosis was 2.1 (0.24–18.4) in survivors and 2.5 (0.45–11.3) in nonsurvivors of another study. UPC was increased in 78% of dogs, but the nature of the proteins as glomerular or tubular was not studied further [255]. High‐level proteinuria based on UPC is occasionally found in dogs with azotemic leptospirosis, but prominent glomerular involvement is usually lacking as this is predominantly a tubulointerstitial disease. The UPC was a mean of 2.59 ± 2.79 (0.2–9.3) in 6 of the 10 dogs with leptospirosis in which it was measured in one study; most of the dogs were azotemic. The increased excretion of LMW proteins (<69 kD) in all 10 dogs with leptospirosis likely accounted for the increased UPC, as LMW proteins comprised 72.1% of the total urinary proteins measured. A unique band of HMW protein (80–90 kD) was seen in 6 of 10 dogs with leptospirosis, and HMW immunoglobulins were identified in 3 of 10 of these dogs, indicating glomerular origin to urinary proteins in some instances. Albuminuria was not specifically measured [256]. Acute glomerular injury is less commonly the cause of AKI and renal proteinuria in dogs or cats compared to acute tubulointerstitial disease. Rapidly progressive glomerulonephritis (RPGN) often associated with Borrelia positive serology, cutaneous and renal glomerular vasculopathy (CRGV) and hemolytic uremic syndrome (HUS) are associated with predominantly glomerular lesions, renal proteinuria, and AKI in dogs that are discussed in detail below. Based on the nature of these glomerular lesions, glomerular origin proteinuria is expected, but acute tubular necrosis (ATN) is sometimes a component of these diseases, so tubular proteinuria may also be documented. Active acute GN is rarely recognized in veterinary medicine, but a syndrome of RPGN (or ICGN) has been described. Borrelia infection associated with chronic glomerular disease and proteinuria was initially reported in one dog [257]. RPGN has been reported mostly in young dogs in Lyme endemic areas. Less than 2% of Lyme seropositive dogs develop this aggressive form of Lyme nephritis, and 9–28% of affected dogs have a history of concurrent or prior lameness [258]. Nearly all dogs with RPGN are Borrelia seropositive, but coinfections with other tick‐borne organisms may also be encountered with Ehrlichia, Anaplasma, Babesia, Bartonella, other Borrelia spp., Rocky Mountain spotted fever, heartworm (HW), or leptospirosis [259]. Membranoproliferative GN was the most commonly documented renal lesion (88%) followed by membranous GN (10%) of affected dogs. Subendothelial deposits of IgG, IgM, and C3 were frequently observed within the glomerulus [218]. The antigen in the immune complexes has not yet been identified, and the relationship of Borrelia seropositivity and the development of RPGN is not clear [259]. The combination of GN with tubular necrosis and regeneration along with interstitial inflammation are distinctive and unique lesions of RPGN. Silver staining of renal tissue may identify the presence of small numbers of spirochetes, but this finding is uncommon. The direct invasion of Borrelia into renal tissue does not appear to be a primary driver for the development of RPGN. The necrosis of glomerular tufts, glomerular crescents, periglomerular fibrosis, and fibrinoid necrosis of small‐to‐medium‐sized renal vessels are observed in some cases [218, 258, 260]. Severe proteinuria in dogs with RPGN is often associated with the development of progressive renal failure and hypoalbuminemia, peripheral edema, and body cavity effusions that are fatal; oligoanuria develops in some cases. Evidence for hypercoagulability and thromboembolic disease may be documented in some affected dogs as consequences of the proteinuria and hypoalbuminemia. Hematuria, glucosuria, and cylindruria (granular and hyaline) are also seen in some dogs [218, 258, 260, 261]. Early renal biopsy is advocated in dogs with severe proteinuria, declining serum albumin, and progressive azotemia in order to evaluate for the presence of immune complexes (documentation of electron‐dense deposits on transmission EM and/or positive immunofluorescent glomerular staining) that factor into a decision whether to treat with immunosuppressive agents [212, 258]. Labrador Retrievers, Golden Retrievers, and possibly Shetland Sheepdogs are over‐represented in this form of RPGN [30]. A podocytopathy has been suspected, but not yet confirmed for Golden Retrievers and Labrador Retrievers with “Lyme” nephritis (RPGN) associated with a high C6 Borrelia titer and high‐level renal proteinuria. These two breeds may have more severe disease from an underlying podocytopathy when infected with Borrelia or other infectious agents compared with other breeds. It should be noted that ICGN has been frequently documented in Goldens and Labradors with proteinuria from the International Veterinary Renal Pathology Service (IVRPS) database without a specific diagnosis of Lyme disease (Ciancello, personal communication, 2019). Other breeds with documented ICGN related to infectious diseases or drug sensitivities include American Foxhounds, Basenjis, Doberman Pinschers, German Shepherd Dogs, and SCWT [262]. A complement C3 deficiency may cause a secondary GN in the Brittany [262]. CRGV was originally described in the USA as a disease of unknown etiology involving the skin and kidneys of affected kenneled and racing Greyhounds, a syndrome initially called Alabama Rot [263]. This syndrome has recently been extensively reviewed [264]. Acute disease of small blood vessels in the skin and kidneys of dogs appears to uniquely define CRGV [265]. Ulcerated skin lesions without systemic signs occurred with most frequency in an early report. Skin lesions in association with azotemic CKD occurred in 44 of 168 dogs in one study. Glomerular necrosis and coagulation within the glomerular capillaries were distinctive lesions in eight dogs that underwent necropsy. The afferent glomerular arteriole was more affected than the efferent arteriole. Hyaline, granular, and RBC casts were observed within some renal tubular lumens. Some of the RBC casts contained fragmented RBC. Thrombocytopenia and anemia were described in some dogs [263]. At the time of CRGV diagnosis in another series of 18 racing Greyhounds, 10 were azotemic, 16 were anemic, 11 were hypoalbuminemic, and all had thrombocytopenia. None of the azotemic dogs in this series survived (8 of 10 were anuric or oliguric); seven of the eight nonazotemic dogs survived. UPC was ≥1.0 in two of six nonazotemic dogs and five of five azotemic dogs (1.19–7.0) [266]. Median UPC was 0.85 (0.56–1.14) and 3.42 (1.81–7.64) in nonazotemic and azotemic dogs, respectively, in another study [267]. Over 95% of the azotemic AKI dogs had occult blood (hemoglobin or myoglobin) on their urine chemistry dipstrip, and about 1/3 had glucosuria and some had hyaline or granular cylindruria [267]. Changes in glomerular endothelial cells were prominent during EM of 12 Greyhounds with CRGV that had some combination of characteristic ulcerated skin lesions, azotemia, and thrombocytopenia [268]. Many different breeds of dogs in the UK were found to be affected with CRGV, in contrast to only greyhounds in the USA [265, 269]. Working dogs (hounds and gun dogs) were at increased risk compared to terriers; no toy breeds were reported. Females and neutered dogs were at increased risk, and seasonal outbreaks of this disease were characteristic [269]. Most dogs of one report were initially evaluated for a skin lesion(s) followed by signs of systemic illness. Nearly all dogs (26 of 30) were azotemic at the time of diagnosis and the remaining four developed azotemia shortly thereafter. In 17 dogs with a UA, the mean USG was 1.020, occult blood positive in 16, protein positive on dipstrip in 11, and glucose positive in 6. It was not determined if the occult blood reactions were from hemoglobin in RBC or from myoglobin. The median UPC was 5.6 with a range of 1.35–16.6 in 11 dogs in which it was measured. Moderate‐to‐severe thrombocytopenia was common. Thrombotic microangiopathy (TMA) was the characteristic glomerular lesion along with fibrinoid necrosis of glomerular arterioles. Endothelial cell hypertrophy and swelling were common (17 of 30). Tubular lumens were frequently filled with protein casts and sometimes with RBC casts. Glomeruli were distended with RBC, some of which were fragmented. Lesions of ATN were identified in 29 of 30 dogs, presumably secondary to renal ischemia from glomerular thrombosis. Twenty‐four dogs either died or were euthanized due to the extent of their disease; an additional six were euthanized due to owner concerns about prognosis or financial constraints. Oligoanuria and progressive azotemia were the most common reasons for death or euthanasia in this study [265]. In azotemic CRGV dogs of another study in which urine output was measured, oliguria occurred in 30/61 and anuria in 13/61 [264]. HUS has been described in a small number of dogs with azotemic AKI that is often preceded by hemorrhagic diarrhea. HUS is defined by the combination of azotemic AKI, thrombocytopenia, and microangiopathic hemolytic anemia. Abnormal RBC morphology from shearing has been described, as well as hemoglobinemia and hemoglobinuria [270–272]. Renal proteinuria in HUS is expected as described above for CRGV due to the severity of glomerular lesions. In one dog with a silent urinary sediment, the occult blood reaction was 4+ and protein 3+ on dipstrip chemistry in association with a USG of 1.027. The occult blood reaction is presumed to be from hemoglobinuria. The proteinuria reaction could result from a combination of renal proteinuria and prerenal protein measured in the filtered hemoglobin [271]. In another case with a USG of 1.018, the dipstick chemistry was positive for occult blood and protein, but it was not characterized further. It has not been determined if HUS and CRGV are variants of a disease with the same cause or not. HUS in dogs has not been described in association with skin lesions, whereas characteristic skin lesions are commonly encountered in dogs with CRGV [264, 265]. TMA is a characteristic lesion common to both CRGV and HUS. Definitive diagnosis requires identification of TMA on renal histopathology. TMA is characterized by vascular inflammation and microthrombi of small vessels that result in ischemia, necrosis, and infarction [270–272]. Consumptive thrombocytopenia, hemolytic anemia with red cell shearing, and multiple organ disruption can also be part of this pathology [264, 265,273–275]. TMA is an uncommon renal lesion as it was encountered in <1% of renal biopsies evaluated by the IVRPS. Collagen type 3 glomerulopathy (collagenofibrotic glomerulopathy) was described in a mixed‐breed family of dogs. This glomerular disease was characterized by a massive accumulation of collagen type 3 in the glomeruli. This disease appears to be inherited in a simple autosomal recessive pattern, but the specific gene defect was not identified [276]. Progressive high‐magnitude proteinuria, systemic hypertension, azotemia, and NS developed in four of seven puppies following cross‐mating of parents that had previously produced affected puppies [277]. Four of nine Drever puppies developed this glomerulopathy at a young age as confirmed by EM and immunohistochemistry. Collagen type 3 predominated, but there was also the presence of collagen type 5 and rare collagen type 1 [278]. A similar disease was described in three of eight Newfoundland dog littermates with severe proteinuria, hypoalbuminemia, and progressive azotemic CKD. Mesangial glomerulosclerosis and glomerular fibrosis were prominent histopathological findings on light and EM. Collagen fibrils within the GBM, within the mesangium, and below the visceral epithelium were described. Lesions of glomerulosclerosis were far more severe than in other familial nephropathies in dogs. It was proposed that a metabolic defect of endothelial or mesangial cells could result in collagen formation. Immunochemistry to confirm the presence of collagen type 3 was not performed [279]. An autosomal dominant glomerular disease associated with proteinuria and a variable rate for progression of CKD has been described in Bull Terriers [280, 281] and Dalmatians [282]. Males and females were equally affected in both breeds, and the GBM was thickened, lamellated, vacuolated, and displayed some subepithelial frilling on ultrastructure. Similar changes on EM are described as in those with HN, but with normal immunostaining of collagen type 4 side chains. The gene mutation leading to abnormal synthesis of GBM proteins or collagen has not been identified [281, 282]. Familial renal disease has been described in Doberman Pinschers [283–285], Beagles [286], and Rottweilers [287] in association with renal proteinuria, progressive CKD, and electron micrographic changes within the GBM similar to that observed in HN, but the genetics and type 4 collagen composition have not been evaluated [221]. There is currently no genetic test available to screen for HN in these breeds [30]. In Doberman Pinschers, mesangioproliferative GN [285] or glomerulosclerosis [284] was described as the underlying light microscopic glomerular lesions associated with familial nephropathy, but the method of inheritance was not determined. In another study, the EM of renal tissue from eight Dobermans affected with juvenile renal disease and membranoproliferative GN by light microscopy was evaluated. The lamellation of GBM was identified as one distinct lesion (five of eight dogs). The accumulation of cross‐banded fibers likely to be non‐type‐4 collagen within the GBM was identified (three of eight dogs) as a second distinct ultrastructural lesion in this study, but the nature of the collagen was not further characterized. These lesions suggested that the glomerulus was damaged as a result of a metabolic defect in collagen synthesis or metabolism [283]. Ultrastructural lesions of what appears to be primary glomerular fibrosis in these Dobermans are similar to those reported in Newfoundland [279] and in dogs with collagen type 3 glomerulopathy [277]. A progressive familial nephropathy characterized by glomerular proteinuria and azotemia has been described in French (Bordeaux) Mastiffs. It appeared likely that this was an autosomal recessive genetic disorder. EM did not reveal splitting or lamellation of GBM as described for HN but did show nonspecific changes featuring podocyte foot process fusion; no immune complexes were identified. Light microscopy revealed changes compatible with membranoproliferative GN [288, 289]. Bull Mastiffs appear to have an autosomal recessive familial nephropathy with light microscopic changes similar to that seen in French Mastiffs [288]. This disorder is characterized by proteinuria associated with glomerular lesions, but further evaluation with EM was not performed [290]. Familial nephropathy was described in three of nine Bedlington Terrier puppies from the same litter with advanced CKD, but the protein content of urine was not reported [291]. Membranoproliferative GN associated with glomerular proteinuria and progressive renal disease has been described in Bernese Mountain dogs as an autosomal recessive disorder [292, 293]. Immune complexes associated with IgM and complement were frequently identified, but an antigen was not identified. EM showed subepithelial electron dense deposits and duplication of GBM [293], but not splitting of GBM as seen in HN. A progressive familial CKD in association with isosthenuria and proteinuria affecting young Golden Retrievers has been reported as juvenile renal disease [294] or renal dysplasia [295]. Cystic glomerular atrophy and periglomerular fibrosis were extensive and fetal glomeruli were observed in some of these dogs. The mode of inheritance was not established nor was an ultrastructural study performed. Familial renal disease was suspected in Norwegian Elkhound dogs of both sexes based on analysis of pedigree in an early report, but the mode of inheritance was not established. Isosthenuria or hyposthenuria was present in all nine dogs. Moderate proteinuria by dipstrip (2+ or 3+) was seen in three dogs, trace in two dogs, and zero in three dogs. Glucosuria was noted for some dogs and aminoaciduria in one dog. Histopathology showed advanced changes of fibrosis and cystic glomerular atrophy along with thickening of Bowman’s capsule, and some glomerular adhesions to Bowman’s capsule. Fibrosis was accompanied by minimal lymphoplasmacytic inflammation [233]. Over one‐third of dogs from a specific line of inbred Norwegian Elkhounds were diagnosed with renal disease between 4 and 48 months of age in another study, but proteinuria was not detailed; aminoaciduria was detected in a few dogs [296]. Renal biopsy at 3–14 months of age from this same line showed periglomerular fibrosis as the earliest renal lesion, a finding that developed prior to the onset of azotemia and urinary concentrating defects. Nephron number and size were normal during early study of these Norwegian Elkhounds, which does not support a diagnosis of renal cortical hypoplasia [222]. Abnormality in genes coding for collagen type IV side chains (alpha 3 and alpha 4) were not found in one study [297]. Renal dysplasia results from disorganized development and defective differentiation of renal tissues. Histopathology reveals various combinations of asynchronous differentiation of nephrons, persistent mesenchyme, persistent metanephric ducts, atypical tubular epithelium, and dysontogenic metaplasia. Lhasa Apsos and Shih Tzus were overrepresented in one large series of dogs. Many abnormalities of glomerular structure exist in those with renal dysplasia [298] so the finding of renal proteinuria is expected. Proteinuria, isosthenuria, and progressive CKD were described in Shih Tzu dogs with renal dysplasia due to a suspected simple recessive mode of inheritance in one study. The presence of fetal glomeruli was the most consistent finding; no ultrastructural evaluation was performed [299]. In an earlier study of young Lhasa Apso and Shih Tzu dogs with progressive CKD, proteinuria was not a consistent feature. No cause or specific renal lesion was identified though the presence of fetal glomeruli was common. The evaluation of renal tissue was limited to light microscopy. The mode of possible inheritance was not determined in this study, but a pilot study indicated that it was not a simple autosomal recessive disorder. Variable proteinuria was reported in this small number of dogs [300]. A familial renal disease was suspected in two litters of related Standard Poodles. Isosthenuria and proteinuria were detected when urinalysis findings were available from a small number of dogs. Immature glomeruli and collapse of capillary lumens were seen on light microscopy suggestive for renal dysplasia. Ultrastructural evaluation was not performed, and the mode of possible inheritance was not further investigated [301]. Isolated cases of renal cortical hypoplasia were described in a single Malamute puppy (protein not reported on urinalysis) [302] and in a litter of Keeshonds with proteinuria and advanced CKD [303]. There are many other sporadic case reports of CKD in young dogs associated with proteinuria, but most of these have not been well characterized as to a likely genetic origin or specific lesion leading to proteinuria. Not all breeds with a familial predisposition for renal proteinuria will have proteinuria attributed to a genetic defect. Consequently, it is important to also evaluate these patients for the possibility of ICGN and glomerular amyloidosis (Rachael Cianciolo, personal communication, 2019). Renal amyloidosis results in variable amounts of amyloid fibril deposition in the glomerulus and interstitium (Figures 7.12 and 7.13) and can be either genetic or acquired. Amyloidosis is a less common cause for PLN in dogs than GN, affecting 7–23% of dogs with biopsy proven glomerular lesions [60, 74, 304]. Most dogs and cats have a reactive form of renal amyloidosis, which develops secondary to chronic inflammation or neoplasia and stimulation of acute phase proteins [305–311]. Animals with substantial glomerular deposits of amyloid will have moderate‐to‐severe proteinuria, whereas those with mostly interstitial deposits have minimal to no proteinuria. In one series of dogs with histopathologic evidence for glomerular disease, the magnitude of increased UPC, severity of hypoalbuminemia, and frequency of azotemia were greater in those with amyloidosis compared to those with GN [304]. In another study, mean urinary protein excretion was highest for dogs with renal amyloidosis, but there was overlap in the ranges between these values in dogs with GN and those with glomerular atrophy making a definitive diagnosis impossible based on the magnitude of proteinuria alone; renal biopsy was required to make these distinctions [205]. Acute renal failure sporadically happens in dogs and cats with renal amyloidosis due to papillary necrosis [312, 313], presumably due to medullary deposits contributing to medullary hypoxia. FIGURE 7.12 Extensive glomerular amyloid in a dog with PLN and azotemia; H&E. Note the homogeneous eosinophilic (pink) extracellular amyloid material and hypocellularity of the glomerulus in the center of this field. Source: Courtesy of Dr. Steven DiBartola, The Ohio State University CVM. FIGURE 7.13 Glomerular amyloidosis. Note the green birefringence within the glomeruli as viewed under polarized light, after Congo red staining. Source: Courtesy of Dr. Stephen DiBartola, The Ohio State University CVM. Beagles, Collies, and Walker Hounds were found to be at increased risk for development of renal proteinuria due to amyloidosis [305]. A hereditary predisposition for reactive renal amyloidosis diagnosed during middle age was suspected in related beagles [314] and in related English foxhounds [312]. Beagles and English foxhounds had both glomerular and interstitial amyloid fibril deposits, in addition to proteinuria and CKD. The mode of inheritance was not determined. Familial secondary renal amyloidosis has been described in related Chinese Shar‐Pei dogs; mean age at diagnosis was 4.1 years. All of the dogs in one study had medullary amyloid deposits, whereas 64% had glomerular involvement. Moderate‐to‐severe proteinuria was apparent in most of these dogs; two dogs with only medullary amyloid had zero to trace proteinuria. The histopathology in Chinese Shar‐Peis was similar to that described for Abyssinian cats (Figure 7.14) [315]. Between 25% and 50% of Shar‐Pei littermates are expected to develop renal amyloidosis if one parent had renal amyloidosis or a history of recurrent fever of unknown origin (Shar‐Pei fever). It appears that this disorder is autosomal recessive and affects Shar‐Pei dogs at an earlier age than non‐Chinese Shar‐Pei dogs [316]. FIGURE 7.14 Amyloid material in glomerulus from an Abyssinian cat with CKD and proteinuria; Congo Red. Congo Red positive uptake supports the diagnosis of glomerular amyloid. Source: Courtesy of Dr. Stephen DiBartola, The Ohio State University CVM. Renal proteinuria was described in eight related young‐to‐middle‐aged Bracchi Italiani dogs (median, five years; 2–10 range) diagnosed with familial nephropathy. All of the dogs were proteinuric and four were azotemic at the time of diagnosis. The median serum creatinine was 4.1 mg/dL (range, 0.8–7.8 mg/dL). Serum albumin was below the reference interval in all dogs but did not correlate with the magnitude of the proteinuria; serum cholesterol was increased in one of eight dogs. Clinical signs were variable and nonspecific, with inappetence being the most common in three of eight dogs. The median UPC was 8.3 (range, 1.3–30) in five dogs in which it was measured; dipstrip protein was positive at 2+ to 3+ in the remaining three dogs. The underlying glomerular lesion was glomerular amyloidosis in six dogs, nephrosclerosis in one, and nonamyloidotic fibrillar glomerulopathy in one dog. The prognosis was poor once clinical signs were manifested [317]. Related Abyssinian cats have been described with secondary renal amyloidosis and renal papillary necrosis [308, 313, 318]. Medullary and glomerular amyloid deposits occur together, but medullary deposits can predominate. Some combination of renal proteinuria, minimally concentrated urine, and azotemia leads to the diagnosis. It appears that longstanding proteinuria of glomerular origin can lead to further glomerular injury, tubular damage, tubulointerstitial inflammation, and progressive nephron loss (Figure 7.15) [93, 103, 104, 167, 199, 207,325–328]. Despite a reduced global GFR (mL/min) in CKD, glomerular permeability increases to macromolecules, especially albumin during the early stages of disease. Single‐nephron glomerular capillary hypertension develops as an adaptation to substantial nephron loss in CKD regardless of cause. This then favors increased filtration of plasma proteins across the glomerulus following increases in single‐nephron GFR and transglomerular pressure [23, 329, 330]. Studies in dog and cat models of subtotal nephrectomy CKD support this scheme [105, 106]. It appears that further renal injury is greatest when systolic blood pressure (BP) is >160 mmHg in the dog and cat [331], but renal injury with systolic BP <160 mmHg is still possible in CKD when systemic pressure is transmitted to the glomeruli from failed vasoconstriction of the afferent arteriole [105, 106, 332].
CHAPTER 7
Proteinuria
INTRODUCTION
GLOMERULAR AND TUBULAR HANDLING OF PROTEIN IN HEALTH
Category
Subcategory
Mechanism
Causes/Examples
Comments
Prerenal ‐ Rare
Glomerular barrier function is normal – selectivity maintained for molecular size and charge
Overload of small MW molecules in plasma that can cross the normal glomerulus into urine (overflow)
Myoglobinuria – rhabdomyolysis
Hemoglobinuria – systemic hemolysis
Bence Jones Proteinuria (light chains) Multiple Myeloma or Paraproteinemia (lymphoma)
Lysozymuria (muramidase) – acute monocytic/myelocytic leukemia
Myoglobin has no plasma carrier – clear plasma; high CK supportive
Hemoglobin has a carrier protein that must first become saturated before free hemoglobin can enter urine – pigmented plasma
Postrenal
Urinary
Very Common
Glomerular barrier is normal
Blood loss into urine – capillary bleeding or inflammation
Ureter, bladder, urethra
Trauma, Cystocentesis, Urolithiasis, Urothelial neoplasia, Urinary infection
Cystitis (including interstitial) urethritis, urethral obstruction, ureteral obstruction
History – LUT urgency or trauma; physical exam, imaging, urine culture, urine cytology, histopathology
Genital
Occasional
Glomerular barrier is normal
Blood loss into urine
Prostate, penis, prepuce, uterus, vagina vestibule, vulva
Semen ‐ normal intact males
Prostatitis, prostatic neoplasia, benign Prostatic hypertrophy (BPH)
Estrus, pyometra, metritis, balanoposthitis, TVT
Mating, ejaculation, normal retrograde urine flow
History, physical exam, imaging, urine cytology, histopathology
Renal
Functional
No structural renal lesions. Transient dysfunction of the glomerular barrier results in mostly albuminuria. Renal vascular congestion and adrenergic system activation result in proteinuria
Fever, seizures, exposure to extreme heat or cold, strenuous exercise (nonconditioned dogs), congestive heart failure, large tumors obstructing blood return from vena cava
UPC often <1.0
History, physical exam, thoracic and abdominal imaging, echocardiogram
Transient nature of proteinuria
Pathological
Result of functional or structural lesions within any region of the kidney
Proteinuria is persistent
Glomerular
Abnormal glomerular barrier
Loss of glomerular permselectivity
Systemic hypertension
(any cause)
Primary glomerulonephritis, hereditary nephropathy or glomerulopathy, glomerulosclerosis (CKD), amyloidosis
Secondary glomerulonephritis: chronic infectious or parasitic disease, acute and chronic inflammation, neoplasia, immune‐mediated disease, drug reactions, leptospirosis (rarely), any disease with circulating chronic antigen excess (immune complexes)
Exogenous glucocorticosteroid,
(D), Cushing’s disease (D), hyperthyroidism (C), diabetes mellitus, critical illness
UPC often >0.5–1.0
UPC > 1.0 and often >2.0 at time of diagnosis
Tubular
Glomerular barrier is normal
Tubulopathy:
Decreased tubular reabsorption of
small MW molecules:
α1‐microglobulin
β2‐microglobulin
Retinol‐binding protein
Lysozyme
Some moderate MW molecules (albumin)
Increased tubular secretion (THP)
Decreased THP CKD
Leakage of intracellular or luminal tubular cell proteins (enzymuria; GGT, NAG)
AKI, ATN, ARF
Leptospirosis
Fanconi syndrome – congenital (D) – Basenji
Fanconi syndrome – acquired: jerky treats (D), drugs; sometimes part of AKI; Cooper associated hepatitis (D); chlorambucil (C)
CKD – tubules fail to reabsorb small amounts of albumin and nonalbumin proteins that are normally reabsorbed by proximal tubules
CKD – proximal tubular reabsorptive capacity exceeded by glomerular leakage of plasma proteins
UPC < 2.5
UPC often 0.5–1.0
Glucosuria and aminoaciduria if generalized proximal tubulopathy
Interstitial
Glomerular barrier is normal
Exudate or plasma enters tubular lumens from peritubular capillaries
Allergic drug reactions
(acute interstitial nephritis)
Leptospirosis
Pyelonephritis (bacterial)
Tubules and interstitium are often involved with the same disease process at the same time
Tubulo‐interstitial disease is the term often used
LOCALIZATION AND CLASSIFICATION OF PROTEINURIA
PRERENAL PROTEINURIA
POSTRENAL PROTEINURIA
RENAL PROTEINURIA
Glomerular – Primary
Glomerulonephritis
Immune‐mediated disease
Anti‐GBM disease (rare)
Familial/hereditary nephropathy (D)
Juvenile renal disease (D)
Familial GN – sibling cats
Minimal change glomerular disease
Idiopathic hypertriglyceridemia
Amyloidosis
Glomerulosclerosis
Glomerular – Secondary
Systemic hypertension – most secondary to CKD; idiopathic is rare
Endocrine
Diabetes mellitus
Hyperadrenocorticism (D)
Hyperthyroidism (C)
Acromegaly (C)
Exogenous glucocorticosteroids
Critical illness
Acute pancreatitis
Pancreatic fat necrosis (C)
Sepsis
SIRS
Pyometra (D)
Immune‐mediated
Immune‐mediated hemolytic anemia (IMHA)
Immune‐mediated thrombocytopenia (ITP)
Immune‐mediated polyarthritis (IMPA)
Systemic lupus erythematosus (SLE)
Infectious
Vector‐borne diseases (D)
Anaplasma, Babesia, Bartonella, Borrelia burgorferi, Ehrlichia, Hepatazoon americanum, Leishmania, Rocky Mountain Spotted Fever
Leptospirosis
Endocarditis
Brucella canis (D)
FeLV, FIV, FIP, Morbillivirus (C)
Canine adenovirus type 1 – Hepatitis (D)
Mycoplasma spp (D)
Mycoplasma gatae (C) chronic progressive polyarthritis
Blastomycosis (D)
Histoplasmosis (D)
Coccidiomycosis (D)
Chronic bacterial infections
Chronic pyoderma
Chronic prostatitis (D)
Parasitic
Heartworm disease (dirofilariasis)
Trypanosomiasis
Schistosomiasis (Heterobilharzia americana)
Cytauxzoon felis (C)
Neoplastic
Lymphoma
Mast cell tumor
Osteosarcoma
Polycythemia rubra vera (primary erythrocytosis)
Functional
Fever, seizures, passive renal congestion, CHF, temperature extremes, exercise (severe in the nonathlete)
Miscellaneous
Cyclic hematopoiesis (Gray Collie syndrome)
Renal thromboembolic disease
High dietary phosphate (C)
Tubulo‐interstitial
Fanconi syndrome
Congenital: Basenji (D)
Acquired: drugs, jerky treats (D)
Chlorambucil (C)
Leptospirosis (D)
Pyelonephritis
AKI/ARF: nephrotoxins, renal ischemia
Allergic drug reactions (interstitial nephritis)
DEVELOPMENT OF RENAL PROTEINURIA
MEASUREMENT OF PROTEINURIA
DIPSTRIPS
Positive reaction
False positive reaction
Negative reaction
False negative reaction
Albuminuria ≥30 mg/dL
(Trace = 10–20 mg/dL)
Most sensitive to albumin
Less detection of nonalbumin proteins
Cauxin (cats)
>1.050 USG trace or 1+ (protein concentration increased by water removal from urine)
Blood contamination – if pink or red urine (plasma proteins and hemoglobin)
Highly alkaline urine (pH ≥ 8.5)
Highly pigmented urine
Blood substitutes (Oxyglobin®)
Phenazopyridine (urinary analgesic)
Disinfectants
Chlorhexidine (Skin cleanser)
Quaternary ammonium (compounds)
Oversaturation of pad dilutes buffer – prolonged immersion
Pad exposure to air and moisture – loss of reagent or reactivity from improper storage
pH pad runover to adjacent protein pad
Semen
Albumin <30 mg/dL (microalbuminuria)
Non albumin proteins
may or may not be detected:
Myoglobin
Hemoglobin
Immunoglobulins
Bence Jones Proteins
Acute Phase Proteins
Mucoproteins
Tamm–Horsfall (uromodulin)
Pad contaminated by finger touching
Pad not exposed to enough urine volume
Low urine pH
Very dilute urine
PRECIPITATION TESTING FOR URINARY PROTEINS
URINARY PROTEIN‐TO‐CREATININE RATIO
Urine protein/urine creatinine ratio (UPC)
Classification
<0.2 (dogs)
<0.2 (cats)
Nonproteinuric
0.2–0.5 (dogs)
0.2–0.4 (cats)
Borderline proteinuric
>0.5 (dogs)
>0.4 (cats)
Proteinuric
MICROALBUMINURIA
URINARY PROTEIN ELECTROPHORESIS
INTERPRETATION AND MONITORING OF RENAL PROTEINURIA
Dipstrip
SSA
UPC
MA a
Interpretation
0
0
<0.2
Neg
No proteinuria
0 or trace
0
<0.2
Positive
Albuminuria
Mild or early proteinuria
Trace to 1+
0
<0.2
Neg
No proteinuria
Trace to 1+
0 to 1+
>0.4(c)
>0.5(d)
Neg
False‐negative MA – uncommon
Tubular proteinuria – most Likely
1+
1+
<0.2
Positive
Albuminuria – mild
Trace to 2+
0
<0.2
Negative
False‐positive dipstrip
2+
2+
0.2–0.4 (c)
0.2–0.5 (d)
Positive
Albuminuria
Mild‐to‐moderate proteinuria
3+
2+
>0.4 (c)
>0.5 (d)
Positive
Albuminuria
Moderate‐to‐severe proteinuria
3+
3+
>2.5
Positive
Severe proteinuria
Primary glomerular disease likely
Protein‐losing nephropathy
Rule out nephrotic syndrome – Evaluate serum albumin and clotting cascade
DISORDERS CHARACTERIZED BY RENAL PROTEINURIA
GENETIC DISORDERS
HEREDITARY NEPHROPATHY
PODOCYTOPATHY
IMMUNE COMPLEXES, GLOMERULAR DISEASE, AND RENAL PROTEINURIA
ACUTE KIDNEY INJURY
OTHER FAMILIAL GLOMERULOPATHIES
RENAL DYSPLASIA
RENAL AMYLOIDOSIS
CONSEQUENCES AND PROGRESSION OF GLOMERULAR PROTEINURIA
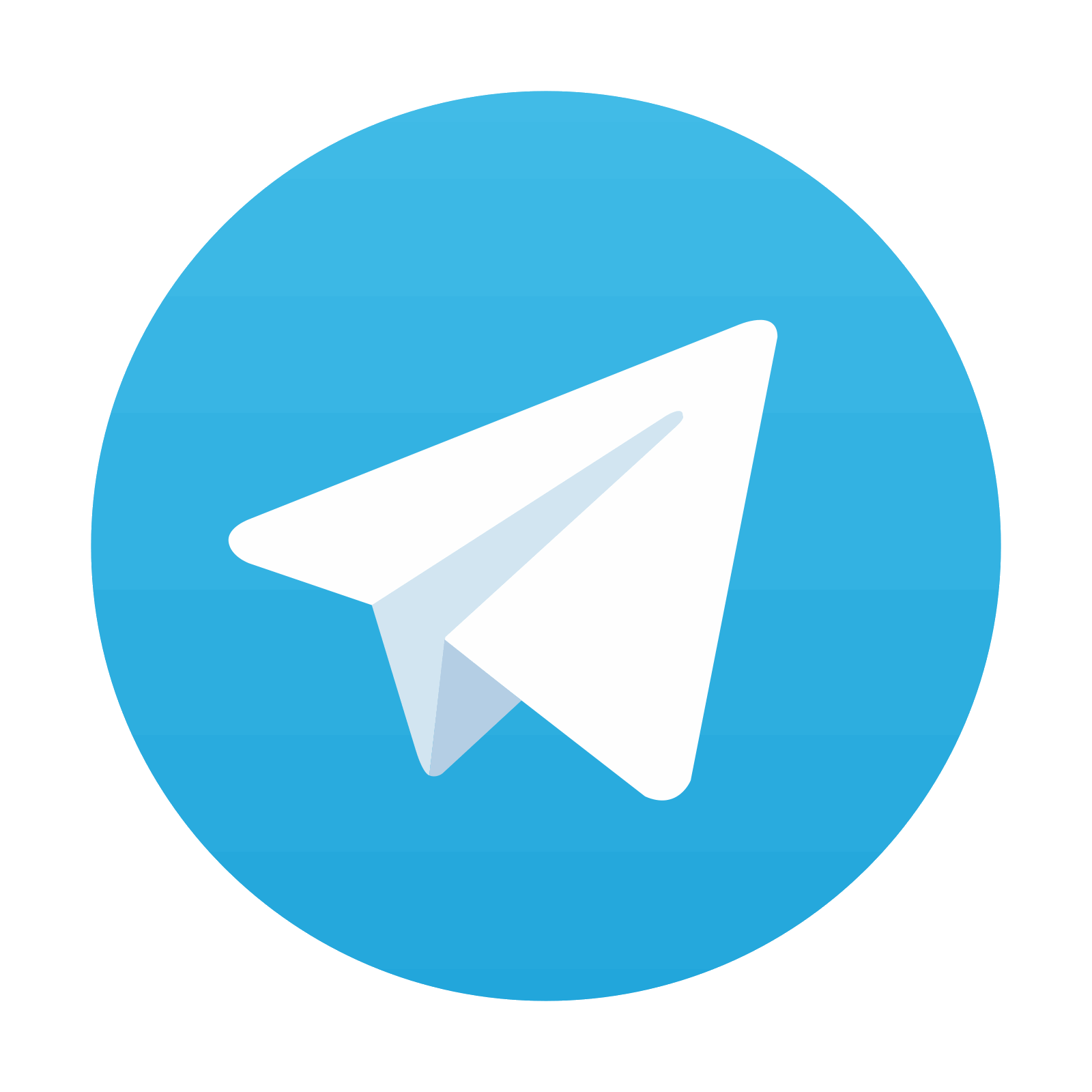
Stay updated, free articles. Join our Telegram channel
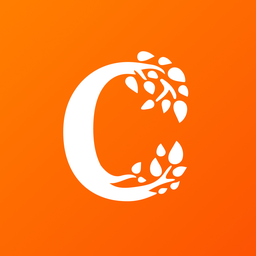
Full access? Get Clinical Tree
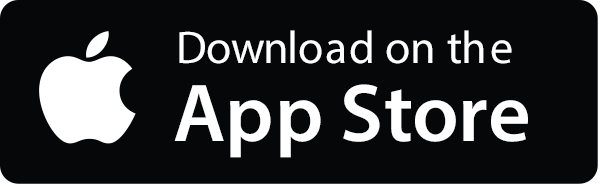
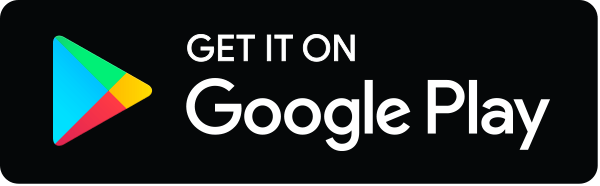