Julie De Backer1 and Shaine A. Morris2 1 Ghent University Hospital, Ghent, Belgium 2 Texas Children’s Hospital and Baylor College of Medicine, Houston, TX, USA The clinical and genetic spectrum of genetic diseases associated with aortic/arterial aneurysm and dissection has expanded dramatically over the past two decades. Nowadays, the term heritable thoracic aortic disease (HTAD) is commonly used to group these disorders. Both syndromic and nonsyndromic HTAD entities exist with overlapping clinical and genetic features. Connective tissue refers to a complex structure or network present throughout the body, which is composed of cells (fibroblasts, smooth muscle cells) and the extracellular matrix (ECM). In addition to its well‐established structural role, connective tissue is known to be a key player in signal transduction and in maintaining tissue homeostasis in many organ systems, including the cardiovascular system. The ECM is a complex and highly heterogeneous structural entity. An important function of the ECM is to support and connect tissues and organs of multicellular organisms, but it is also a highly dynamic structure with diverse roles [1]. It can transmit specific environmental signals to cells, influencing their development, proliferation, migration, polarization, and metabolic functions. The ECM is also pivotal in regulating spatiotemporal bioavailability of growth factors and cellular signaling. Well‐known examples are the ability to store transforming growth factor beta (TGF‐β) and bone morphogenic protein (BMP) 7 [2,3]. The constituents of the ECM are produced by cells specific to the tissue type. For instance, in the aorta they are synthesized by endothelial cells, smooth muscle cells, and fibroblasts. Variations in the amounts of different macromolecules and in their organization give rise to tissue‐specific properties. For example, in the aortic media, concentric layers of elastic fibers provide extensibility, whereas in the eye parallel bundles of fibrillin‐containing microfibrils enable anchorage of the lens to the ciliary body. Although ECM molecules are diffusely present in the endocardial and myocardial layers, the major sites of deposition of cardiac connective tissue are at the fibrous annulus of the semilunar and atrioventricular valves. Also, the leaflets are composed of different layers of connective tissue, covered by endothelial cells. The adult human aortic valve leaflets, for example, are composed of around 10–15% elastin and 45–55% collagen [4]. The composition of the ECM in blood vessels varies between different types of blood vessels (elastic or muscular arteries; small, medium, or large veins). Within blood vessel wall layers (tunica intima, media, and adventitia), the ECM is composed of different molecules, each playing a specific role in maintaining structural and functional homeostasis. Although it is beyond the scope of this chapter to review in detail, the following discussion provides basic pathophysiologic concepts, focusing on those molecules relevant to cardiovascular disease. The paradigm connective tissue disorder (CTD) with cardiovascular involvement is Marfan syndrome (MFS); a detailed description of the cardiovascular features is provided in the next section. Although the first clinical recognition of the disease dates back to more than a century ago, it was not until the early 1990s that the principal protein, fibrillin‐1, and shortly thereafter the causal defect in the fibrillin‐1 gene (FBN1) were identified [5–7]. The fibrillin‐1 protein, identified through electron microscope studies, is a microfibril that contains small‐diameter fibrils in the ECM located close to the basement membranes and also at the periphery of elastic fibers. Fibrillins are glycoproteins and were found to be long, flexible molecules. Other proteins were identified as microfibril‐associated proteins and, overall, these molecules constitute the “fibrillin microfibril scaffold.” From an evolutionary perspective, fibrillin predates all other microfibril proteins and was initially attributed a primarily structural role. Ongoing biochemical studies of molecular cell–matrix interactions in humans and mouse models of MFS, however, have demonstrated that fibrillin‐1 also performs important functional roles, namely in regulating growth factors and in mechanotransduction. Fibrillins play an important role in regulating TGF‐β bioavailability. The initial concept of TGF‐β regulation was based on the knowledge that the fibrillin‐1 protein is homologous with the family of latent TGF‐β‐binding proteins (LTBPs), which serve to hold TGF‐β in an inactive complex in various tissues, including the ECM [8]. Fibrillin‐1 was shown to bind TGF‐β and LTBPs [9–11]. Based on this observation, it was hypothesized that pathogenic variants in fibrillin‐1 could lead to perturbed sequestration of the inactive TGF‐β complex [12], resulting in the increased TGF‐β signaling observed in several tissues in patients with MFS as well as in murine models. Another important concept derived from the observed TGF‐β overactivation was that this induced constitutively elevated signaling by the angiotensin II (AngII) type I receptor (AT1R) [13], which sparked the concept of using angiotensin receptor blockers for treatment. The concept underlying angiotensin II receptor blocker (ARB) use in MFS is that AT1R‐dependent TGF‐β hyperactivity is considered a main driver of the disease and AT1R antagonism may prevent thoracic aortic disease formation by blunting TGF‐β signaling [14]. A trial with losartan in MFS mice showed a significant reduction of aortic root aneurysm progression and improvement of aortic wall architecture compared to treatment with either placebo or propranolol [14]. A small study in children with severe MFS showed promising preliminary human results [15]. Encouraged by these results, at least 12 randomized trials ensued, recruiting more than 2000 patients in total, spanning all age ranges, with varying comparisons of treatment. The initial results from an open‐label trial in a pediatric age group and also in an open‐label study in adult patients with MFS showed a beneficial effect of a combined treatment with beta‐blocker and losartan [16,17]. The results of a large (n = 604) randomized trial in a young (1 month to 25 years of age) MFS cohort, conducted by the Pediatric Heart Network comparing atenolol and losartan as monotherapy, showed no difference in aortic root growth rate between the two treatment groups, although both demonstrated a significant decline in z‐score, suggesting a potential beneficial effect of both medications [18–20]. In a subsequent trial comparing losartan and atenolol as monotherapy in adults, no differences were observed after 3 years or after a subsequent analysis at 7 years [21,22]. Similarly, the initial beneficial effects of losartan combined with beta‐blockers could not be reproduced in a large double‐blind randomized controlled trial, questioning the synergistic effect of these two drugs [23]. A recent trial in children and young adults compared the addition of a more potent ARB, irbesartan, to the addition of placebo to standard therapy [24]. This study demonstrated that the addition of irbesartan to standard therapy reduced the rate of aortic dilation compared to placebo. No difference was noted between the groups in serious adverse events including dissection. Based on current knowledge and the findings of the trials conducted so far, a number of considerations can be made about medical therapies for MFS: Several attempts have already been made to analyze the findings of the trials conducted so far. A recent Cochrane review assessing the efficacy of beta‐blockers to prevent aortic dissection could not confirm any benefit [27]. A network meta‐analysis indicated that the combination of an ARB and a beta‐blocker resulted in the most effective prevention of aortic root dilation, suggesting that an ARB should be added to the treatment regimen if tolerated [28]. In summary, it is fair to say that, thus far, no definitive conclusion for clinical practice can be drawn. A prospective, collaborative meta‐analysis of all randomized controlled trials of angiotensin receptor antagonists in Marfan syndrome, based on individual patient data, is currently ongoing and it is hoped that it will provide some clear answers [29]. The failure of drug treatment thus far has been a major trigger for ongoing research to unravel the pathophysiology of aortic disease in order to identify better treatment targets. Over the years, both previously mentioned concepts (impaired TGF‐β sequestration and signaling via the AT1R) have been challenged and modified. Studies in an Fbn1 mouse in which the LTBP binding site (the site important for TGF‐β binding) was deleted (Fbn1H1Δ) demonstrated that these mice did not present features of MFS [12], hence refuting the importance of TGF‐β sequestration by fibrillin‐1. In Fbn1C1039G/+ MFS mice lacking the AT1 receptor, losartan did not mitigate the process of aneurysm formation, questioning the direct implication of this pathway and suggesting off‐target effects of ARBs. One intriguing mechanism that has caught recent attention is the nitric oxide (NO) signaling pathway. Based on losartan inactivation by NO synthase (NOS) inhibition and endothelial NOS (eNOS) protection from aneurysm formation in Fbn1C1039G/+ mice [30,31], Sellers and colleagues [31] have concluded that endothelial NO release may mediate this losartan off‐target effect, thus also implying a significant role of endothelial dysfunction in aneurysm progression. NO inhibition is currently being investigated as a potential new therapeutic avenue [32]. Currently the most plausible concept of aneurysm formation in genetic aortic disease is that of altered mechanobiology. According to this model, hemodynamic load imposed on an aortic wall weakened by pathogenic variants in genes encoding ECM components causes improper activation of mechanosensors of vascular smooth muscle cells (VSMCs) and fibroblasts. In response to mechanical stress, VSMCs switch from a contractile to a secretory phenotype and fibroblasts differentiate into myofibroblasts leading to hyperactivation of the TGF‐β pathway, secretion of metalloproteinases and inflammatory agents, increased production of collagen, and elastic fiber fragmentation [33]. Abnormal mechanosensing and mechanotransduction can also lead to impaired endothelial function. Appropriate sensing of mechanical shear wall stress is believed to be essential to properly activate endothelial function through the NO pathway [34]. Figure 33.1 Schematic overview of the concept of mechanobiology underlying homeostasis with indication of components altered by genetic defects in HTAD (in red) and therapeutic targets (in green). Mechanical forces (blood pressure) imposed on the aortic wall lead to cellular and molecular responses. Vascular smooth muscle cells (VSMCs) undergo a phenotypic switch, changing from a contractile to a secretory phenotype. This induces altered secretion of extracellular matrix (ECM) components. Fibroblasts in the aortic adventitia differentiate into myofibroblasts under the impulse of activated transforming growth factor beta (TGF‐β). Endothelial cells regulate vascular function through nitric oxide (NO) regulatory mechanisms. Following its release from the ECM, TGF‐β ligands bind to their receptor. Subsequently both the canonical (Smad dependent) and noncanonical pathway (Erk dependent) are activated and will ultimately lead to the transcription of various TGF‐β‐dependent genes, such as connective tissue growth factor, plasminogen activator inhibitor‐1, and multiple collagens. The ECM also interacts with integrins on the cell surface and subsequently transmits signals to the contractile apparatus consisting of actin and myosin filaments. Alterations, due either to higher imposed forces (hypertension) or to (genetic) alterations in the various components required for proper sensing and/or transduction of the signal may lead to aneurysms/dissections. Molecular components in which mutations lead to aortic aneurysms/dissections are marked in red. AngII, angiotensin II; ARB, angiotensin receptor blocker; AT1R, angiotensin II type 1 receptor; BP, blood pressure; MMP, matrix metalloproteinase; NOS, nitric oxide synthase. Using MFS as a model for this concept, human and mouse studies have revealed that the fibrillin microfibril structure is sensed by the cells (both fibroblasts and VSMCs). These will consequently send a signal through integrins and the cytoskeleton, resulting in inappropriate remodeling and aneurysm formation via a common pathway of inappropriate TGF‐β signaling [35,36]. These concepts were summarized by Sengle and Sakai [37] and Humphrey et al. [33] (Figure 33.1). The involvement of AngII studied in mouse models of various genetic aortic diseases indicates that mechanisms may vary according to the type of defect. In mice deficient in fibulin4 (another ECM component), potentialization of the ACE is observed whereas in an ACTA2 model (with a defect in the VSMC contractile apparatus), AngII activation is induced by NFκb stimulation of specific B cells. Given the distinct nature of the underlying genetic lesion driving aneurysm formation (ECM versus contractile unit impairment), it would be interesting to further unravel if the genes drive the different roles of AngII signaling [38]. Further understanding of these mechanisms is crucial in developing more targeted treatment strategies for HTAD [32]. Heritable thoracic aortic disease comprises a heterogeneous group of disorders associated with aortic aneurysm or dissection potentially involving the area from the aortic root to the diaphragm. Aneurysms and dissections in more distal parts of the aorta and/or other vessels may co‐occur in some HTAD entities. Depending on the presence or absence of manifestations in other organ systems, HTAD can be further subdivided into syndromic and nonsyndromic HTAD. Among the syndromic forms, MFS is the best known and also the best delineated with established diagnostic criteria (see section “Marfan syndrome”). There is notable clinical overlap between the different syndromic entities, and pathogenic variants in practically all the genes leading to syndromic thoracic aortic disease are also identified in patients and families with nonsyndromic forms [39–41]. In view of the difficulty in distinguishing these disorders clinically, it is recommended to perform panel testing of the known genes using next‐generation sequencing techniques. A set of genes was carefully curated in 2017–2018 and gene–disease association was confirmed for a selection of them using a semiquantitative method that can be found in Renard et al. [42]. Consistent with the degenerative nature of thoracic aneurysms and dissections, inherited forms of thoracic aortic disease are accounted for by pathogenic variants in genes encoding molecules normally implicated in supporting tissue integrity and homeostasis, such as components of the ECM, VSMC cytoskeleton, and TGF‐β signaling pathways [43]. An overview of the different clinical entities and their underlying genetic defect is provided in Table 33.1. Echocardiography is commonly used for cardiovascular imaging in CTD. It allows for high‐resolution evaluation of aortic dimensions, pulmonary artery dimensions, mitral and tricuspid valve function, and ventricular size and function. Echocardiography is typically readily available and can be obtained in conjunction with a clinical evaluation. The primary limitation of echocardiography in patients with CTD is restricted acoustic windows, often related to body habitus and postoperative changes. At times, cardiovascular magnetic resonance (CMR) imaging and computed tomographic angiography (CTA), which are not limited by body habitus, are used to evaluate the distal aorta and extra‐aortic vasculature, and to obtain three‐ and four‐dimensional imaging. CMR also allows for high‐quality quantitative assessment of ventricular size and function when ventricular dilation or dysfunction is a concern and allows quantitative assessment of aortic and mitral valve regurgitation (see Chapter 47). Common findings noted on imaging in CTDs are listed in Table 33.2. MFS is an autosomal dominant CTD typically caused by pathogenic variants in the fibrillin‐1 gene (FBN1). Pathogenic variants in other genes including TGFBR1, TGFBR2, TGFB2, TGFB3, and SMAD3 have also been identified in rare cases fulfilling the diagnostic criteria for MFS [49–52]. The diagnosis of Marfan syndrome is based on clinical characteristics, although in cases without ectopia lentis (EL) additional molecular testing with confirmation of an underlying FBN1 pathogenic variant may be helpful. Criteria for diagnosis have been published as the “revised Ghent nosology” [53]. In the absence of a family history for MFS, the diagnosis is confirmed when: In the presence of a family history, the diagnostic criteria are less strict: In these criteria, substantial weight is given to aortic root diameter z‐scores, which underscores the importance of cardiovascular imaging in the diagnostic work‐up. Clinical manifestations in MFS vary widely both within and between families. Severe cardiovascular phenotypes exist with significant atrioventricular valve dysfunction and/or rapidly progressive aortic root dilation in infancy, so called “neonatal MFS” or more accurately “infantile MFS” [54–56]. In classic MFS, cardiovascular manifestations are milder, have a slower rate of progression, and can manifest later in life [57,58]. Table 33.1 Overview of the clinical/genetic entities associated with aortic aneurysms; discriminating features are highlighted in bold HTAD, heritable thoracic aortic disease. Echocardiography plays a key role in the diagnostic work‐up of cardiovascular manifestations of MFS and also in the follow‐up and guidance of treatment. Echocardiography, typically every 6–12 months, is used to follow aortic root dimension to determine timing of root replacement, and to follow mitral valve function and ventricular size and function to determine timing of mitral valve surgery. There are no current recommendations for timing of aortic root surgery in children. However, the adult guidelines are commonly employed, which recommend aortic root replacement when the maximum aortic root dimension reaches 5.0 cm, or at 4.5 cm if there is a family history of aortic dissection at diameters less than 5.0 cm, rapidly expanding dilation (see the next section), or presence of significant aortic valve regurgitation. Silverman et al. demonstrated that a family history of severe cardiovascular complications in MFS is associated with increased aortic diameter and decreased survival [59]. Increased risk of aortic dissection at a smaller dimension in MFS is also present in pregnant women and in those with increased vertebral artery tortuosity [60,61]. Table 33.2 Imaging findings noted in connective tissue disorders CMA, cardiovascular magnetic resonance; CTA, computed tomographic angiography. The primary cardiovascular manifestation in MFS is progressive dilation of the aortic root, eventually leading to aortic dissection or rupture. Of note, in this chapter, aortic root describes the aortic segment extending from the aortic annulus to the sinotubular junction, including the sinuses of Valsalva; in certain reports, the aortic root is synonymous with the level of the sinuses of Valsalva. It is estimated that aortic root dilation is present in >80% of children and adult patients with MFS [62–64]. Echocardiographic images are obtained from the parasternal long‐axis view visualizing the aortic root and the proximal ascending aorta (Figure 33.2, Videos 33.1 and 33.2). To optimize the view of the ascending aorta, the long‐axis view is also obtained at a higher intercostal space compared with the conventional long‐axis view. Different transducer positions are often needed to optimize the images: a high left parasternal view located one or two intercostal spaces superior to the standard location may be required. Alternatively, a high right parasternal view in a right lateral decubitus position may better display the entire proximal aorta. Measurements of the aortic root and ascending aorta are taken at different levels: (i) the aortic valve annulus (defined as the hinge points of the aortic leaflets); (ii) the maximal diameter at the sinuses of Valsalva; (iii) the sinotubular junction (transition between the sinuses of Valsalva and the tubular portion of the ascending aorta); and (iv) the ascending aorta at the level of the right pulmonary artery (see Figure 33.2). When measuring aortic diameters, it is important to obtain the maximum diameter recorded perpendicular to the long axis of the vessel in that view. In the pediatric quantification guidelines from the American Society of Echocardiography, the measurement is performed from inner edge to inner edge at end‐systole [65]. This matches the measurements used by other imaging techniques, such as CMR and CTA. In adults, the most recent guidelines from the American Society of Echocardiography recommend measuring at end‐diastole using the leading edge technique [66]. Absolute differences between the two techniques are small and further improvements in image resolution should minimize the difference between these measurement methods [67,68]. Aortic diameter measurements are measured by 2D imaging rather than M‐mode. The latter technique is no longer adequate for aortic root measurements due to motion of the heart during the cardiac cycle and changes in M‐mode cursor location relative to the maximum diameter of the sinuses of Valsalva. This can result in a systematic underestimation (by up to 2 mm) of aortic diameter by M‐mode in comparison with the 2D measurements of aortic diameter [69]. Aortic root dilation in MFS is typically located at the level of the sinuses of Valsalva and is defined as an aortic root diameter above the 95% confidence interval of the normal distribution in a large reference population. Aortic dilation can easily be detected by plotting observed aortic root diameter versus body surface area on previously published nomograms [69]. Another possibility is the use of z‐scores derived from the regression equation on the nomogram. A z‐score ≥2 indicates a value exceeding two standard deviations (the 95% confidence interval) (see Chapter 5). The clinical measurement method should match that used to generate the normative data. For echocardiographic measurements made from inner wall to inner wall during systole in subjects aged ≤25 years, two commonly used z‐score calculators derived from large cohorts can be found at https://www.marfan.org/dx/zscore or http://www.parameterz.com/refs/lopez‐circimaging‐2017 [70]. For echocardiographic measurements made from leading edge to leading edge in diastole in all age groups, reference graphs and z‐score equations are available. Two commonly used equations are those published by Devereux et al. (age ≥15 years) and Campens et al. (all ages) [71,72]. Of note, in children, the measurement of a z‐score of >2.0 is typically referred to as “aortic dilation,” and may be described as mild (usually up to a z‐score of 2.9–3.9), moderate (up to 3.9 to 5.9), or severe (≥4.0 to 6.0) [66,73,74]. The definition of aortic dilation is more variable in adults and has been defined as a z‐score >2, or a measurement >90–95th percentile of expected based on varying algorithms [66,72,75,76]. Although “aortic aneurysm” is not commonly used in children, it is typically defined in adults as >1.5 times the expected diameter based on sex‐based normals [77,78]. However, as this binary definition does not take into account known body size or age associations, many guidelines have elected to use z‐scores instead to guide aortic management [66,78,79]. Figure 33.2 Echocardiographic image of aortic root dilatation at the level of the sinus of Valsalva in a 13‐year‐old boy with a pathogenic variant in TGFB3. (a) Measurements performed according to guidelines for adults at end‐diastole using the “leading edge‐to‐leading edge” principle. (b) Measurements performed according to guidelines for children at end‐systole using the “inner edge‐to‐inner edge” principle. Ao, aorta; LA, left atrium; RPA, right pulmonary artery; RV, right ventricle. In some patients with MFS, aortic root dilation is asymmetric; therefore, measurement in the transverse plane of the aorta (parasternal short‐axis view) may be important. A study by Meijboom et al. [80] using CMR revealed the largest aortic root diameter between the right and left coronary cusps. For a given noncoronary‐to‐right coronary cusp diameter, which is the diameter assessed in the parasternal long‐axis view, the 95% confidence intervals revealed a variation of −20 to +20% in the aortic root area [80]. So far, no echocardiographic study has been performed confirming these results. The predilection for the aortic root and ascending aorta to dilate is a result of both structural and local hemodynamic factors. The aortic root, ascending aorta, and the proximal part of the pulmonary artery (which also dilates in MFS) are derived from embryonic neural crest cells, whereas the more distal arterial structures have a mesodermal origin. The elastic fiber content in the aortic root and ascending aorta has been shown to be higher than in any other parts of the arterial tree [81]. This may explain why diseases that affect elastic fiber integrity have more significant effects in this part of the vascular system. Additionally, the aortic root is subject to the repetitive stress of left ventricular ejection, contributing to progressive dilation [82,83]. It is postulated that since aortic pressures are significantly higher than those in the pulmonary artery, dilation is generally more pronounced in the aortic root. In order to define indications for surgical intervention, absolute growth of the aortic root should be reported in addition to the diameter at any given examination. When following sinus of Valsalva dimensions longitudinally, the expected increase in children on medical therapy is an average of ~0.5–0.8 mm/year, although in a single year this may be as high as 3 mm [20,64]. Z‐scores in children tend to be overall stable, with potential decreases over time with medical therapy [20,84]. For adults, mean sinus of Valsalva growth is slightly lower, ~0.2–0.5 mm/year [85]. Annual growth rates exceeding 10 mm/year in children and 5 mm/year in adults are considered a risk factor for dissection, reflected by the indication for prophylactic surgery [78,86]. Although aortic root growth in MFS is usually followed by echocardiography, in cases of significant dilation in which surgery is being contemplated, an asymmetric aorta, or poor acoustic windows, CMR or CTA imaging may be employed. These modalities allow for 3D evaluation of aortic dimensions. In addition, CMR may provide improved reproducibility compared to echocardiography, although this is most relevant distal to the aortic root [87]. In CMR and CTA imaging, measurement of the aortic root and ascending aorta is recommended to be perpendicular to the long axis of the aorta, employing double oblique methodology [66,88]. Measuring aortic dimensions simply in the axial plane of the chest without using double oblique methods can result in overestimation of the true aortic dimension (Figure 33.3). Figure 33.3 An example of measuring the proximal descending aorta using double oblique methodology on magnetic resonance angiography, ensuring the measurements are perpendicular to the long axis of the aorta. Measurements obtained by CMR/CTA may be up to 2–3 mm larger than those obtained by echocardiography, likely due in part to the ability to image the entire aorta rather than limited 2D views [87,89–92]. Apart from using the double oblique technique, there is currently no consensus on what methodology to use when measuring the aorta, and practice varies greatly [66,88]. These differences in measurement contribute significantly to varying reproducibility between centers when determining an aortic segment measurement [88]. Many research and clinical studies have reported aortic measurements made from inner edge to inner edge on gated or non‐gated images [66]. For the measurement of the sinus of Valsalva, techniques also vary. Different practices may employ sinus‐to‐commissure, mid sinus‐to‐sinus, or maximum sinus‐to‐sinus methods (Figure 33.4). Given this variation in practice, it is critical that the methodology employed is reported, and that the same methodology be used serially. For other aortic segments, it is standard to measure the vessel in a biplane manner (Figure 33.4). The maximum sinus‐to‐sinus method has several advantages, including the ease of detecting cusp margins in short‐axis planes and close agreement with echocardiographic measurements [91,92]. Given this, it has been recommended that, for aortic measurements by CMR and CTA, the three largest sinus‐to‐sinus measurements in end‐diastole at the level of sinus of Valsalva in the short‐axis plane should be averaged. Figure 33.4 Variation in methods to measure the sinus of Valsalva. (a) Sinus to commissure. (b) Mid sinus to sinus. (c) Maximum sinus to sinus. Dissection or rupture occurs as a result of an imbalance between intrinsic wall properties (weakness caused by genetic defects leading to abnormal connective tissue) and mechanical factors (pressure), a process also referred to as altered mechanobiology [93]. This will in most cases first lead to progressive dilation of the ascending aorta with aneurysm formation and, when reaching a critical threshold, to dissection or rupture. Dissection starting in the area extending from the aortic root to the left subclavian artery is referred to as a Stanford type A dissection. Type A dissection may be limited to the ascending aorta or may extend as far as the iliac bifurcation or further. Echocardiography is an important tool in the diagnosis of aortic dissection. It can not only confirm the diagnosis but also identify complications such as pericardial tamponade, aortic valve regurgitation, or acute myocardial ischemia due to coronary artery obstruction or dissection. Although aortic dissection can be diagnosed using transthoracic echocardiography (TTE) with a sensitivity reaching 85% and specificity of 95% [94)], transesophageal echocardiography (TEE), CMR, or CTA are the preferred techniques in older children and adults as they provide better visualization of the ascending aorta, the aortic arch, and the descending aorta (Figure 33.5, Video 33.3). Although most of the arch and origins of two of the great vessels can be seen in most patients, there is a blind area in the upper ascending aorta and the proximal arch that cannot be seen by TEE because of the interposed tracheal bifurcation. For optimal evaluation of the extent of the dissection, CTA is the preferred approach in emergency settings whereas CMR can be used in chronic dissection and for longitudinal surveillance. Figure 33.5 Dissection of the ascending aorta at the level of the sinuses of Valsalva. The arrow indicates the dissection flap. Two‐dimensional echocardiography is the generally used tool for the diagnosis of mitral valve prolapse (MVP). MVP is typically diagnosed in the parasternal long‐axis window as systolic displacement of the mitral leaflet into the left atrium of at least 2 mm from the mitral annular plane (Figure 33.6) [95]. Both parasternal long‐axis and apical four‐chamber views may be used to evaluate MVP (Videos 33.4–33.6) [96,97], although in the four‐chamber view the mitral valve annulus may be visualized along the more basal portion of the saddle‐shaped mitral annulus and may falsely increase the degree of displacement. Imaging from the parasternal short‐axis view is useful for locating the prolapsing leaflet segment(s). 3D echocardiography may be helpful in MVP by providing depth perception. The prevalence of MVP in adults with MFS is estimated between 40 and 68% (compared with 1–2% in the general population) [82,98]. MVP is present in ~32–38% of children with MFS, and prevalence increases with age [99,100]. In the infantile MFS phenotype, severe MVP with moderate to severe mitral valve regurgitation is typically a major feature of the presentation, often accompanied by significant aortic root dilation, left ventricular dilation, possible ventricular dysfunction, and symptoms of congestive heart failure and failure to thrive (Figure 33.7, Video 33.7) [101,102]. Rupture of the mitral valve chordae may also be noted in infantile Marfan syndrome [103]. A study by Rybczynski et al. showed that the incidence of MVP‐related clinical events (endocarditis, surgery, heart failure) in MFS patients was 28% as compared with 13% in patients with idiopathic MVP. The age at event was also significantly lower (35 versus 65 years in idiopathic MVP) [104]. Yetman et al. demonstrated that in a cohort of 70 young patients with Marfan syndrome, all of the 21% with ventricular ectopy, all of the 6% with ventricular tachycardia, and all of the 4% that died of arrhythmia had MVP, usually in association with left ventricular dilation, but not necessarily mitral regurgitation [105]. Figure 33.6 Echocardiographic picture of mitral valve prolapse (parasternal long‐axis window). (a) Image in systole – note the bulging of both mitral valve leaflets into the left atrium (LA). (b) Image in diastole – note the thickened mitral valve leaflets (arrows). AML, anterior mitral valve leaflet; Ao, aorta; LV, left ventricle; PML, posterior mitral valve leaflet; RV, right ventricle. Figure 33.7 Severe mitral valve prolapse in a 14‐month‐old boy with infantile Marfan syndrome. These panels show the elongated and redundant mitral valve (MV) leaflets with several ruptured chordae by transesophageal echocardiography in diastole (a) and systole (b). Note severe aortic root (AoR) dilation and left ventricular (LV) dilation. LA, left atrium. It is unclear if the mechanisms of increased morbidity in MFS noted with MVP are related to the described phenomenon of “malignant MVP.” Malignant MVP is a subset of MVP, usually described in nonsyndromic populations, which appears to be associated with sudden cardiac death [106]. Associations noted with sudden death in populations with MVP that may stratify those with malignant MVP include inverted or biphasic T waves, QT dispersion, QT prolongation, and premature ventricular contractions. Imaging associations with sudden death include a mitral valve leaflet thickness of 5 mm or greater, mitral annulus disjunction, paradoxical systolic increase of the mitral annulus diameter, and fibrosis identified by late gadolinium enhancement on CMR [107]. Although one study has suggested a familial arrhythmogenic form of MVP due to a truncating variant in the FLNC gene, genetic associations with malignant MVP are largely unknown [108]. As improved medical and surgical management has resulted in decreased ascending aortic events in MFS, distal complications have become more concerning [109–111]. These complications include descending aortic aneurysm and acute or chronic dissection. Dissection in the descending aorta may be secondary to a distal dissection starting past the left subclavian artery (Stanford type B dissection), or extension of type A dissection. The prevalence of dilation (defined as z‐score >2.0 standard deviations) of the descending aorta in children who have not undergone aortic surgery and as assessed by CMR is ~50% [112]. Several z‐score calculations exist for the descending aorta in children, including http://www.parameterz.com/sites/aortic‐arch and http://zscore.chboston.org [113–116]. The prevalence of dilation of the descending aorta in adults has been less well characterized, given varying definitions of dilation. A few case reports have described isolated severe aneurysms of the descending aorta in MFS patients [117,118]. Although TTE is capable of imaging some segments of the descending aorta (Figures 33.8 and 33.9), comprehensive evaluation requires CMR or CTA (Videos 33.7 and 33.8). Nollen et al. reported increased growth rate (defined as >1 mm/year) in 6% in the descending thoracic aorta and 7% in the abdominal aorta [119]. They also identified aortic stiffness as an independent predictor of progressive abdominal aortic dilation. Kawamoto et al. studied the progression of thoracoabdominal aortic diameters in patients with MFS after surgical repair and defined a subgroup of patients showing progressive dilation of the distal aorta (>3 mm/year) who may benefit from closer follow‐up [120]. Risk stratification for descending aorta dissection remains poor. A study by Mimoun et al. demonstrated that dissection in the descending part of the aorta may occur independent of the diameter of the ascending aorta [121]. Schoenhoff et al. concluded that previous aortic dissection is a significant risk for recurrent interventions on the untreated aorta, a risk that was more pronounced in those with initial type B dissection compared with type A dissection [122]. Den Hartog et al. reported the most comprehensive assessment of the risk of type B aortic dissection in MFS to date [111]. In 600 patients, the only independent predictors for type B dissection were prior prophylactic aortic root surgery and proximal descending aortic dimension ≥2.7 cm. Notably, 2.7 cm is typically considered the upper level of normal for the proximal descending aorta, and 41% of the cohort met this threshold. About 8–15% of MFS patients require initial surgery in the descending aorta [109,122]. Patients with initial type B aortic dissection are at a significantly higher risk for reintervention (86% for previous type B dissection versus 42% for previous type A dissection). The majority of reinterventions are in patients with previous dissection (48% versus 11% reintervention in the patients presenting with aortic aneurysm) [122]. A large contemporary series of 96 thoracoabdominal aortic aneurysm repairs in patients with MFS showed an excellent early survival rate of 97% [123]. The 2010 guidelines recommend surgical repair at the thoracoabdominal level in MFS when aneurysm diameter exceeds 5.5 cm [78]. Endovascular procedures are not routinely recommended in the setting of MFS or of Loeys–Dietz syndrome (LDS) but may be considered in selected cases to restore perfusion to compromised branch vessels in the setting of acute thoracoabdominal aortic dissection causing abnormal perfusion of vital organs or the limbs [124,125]. Figure 33.8 Recommended echocardiographic windows to assess the diameter of the aorta at different levels. The aortic root is assessed from the parasternal long‐axis view. The ascending aorta is in most cases better viewed one intercostal space higher than the view for the root. Measurements are made at the level of the right pulmonary artery (RPA). The aortic arch is visualized from the suprasternal notch view. The descending thoracic aorta (DTA) can be viewed from the parasternal long‐axis window, located under the left atrium (LA); a longitudinal view of the DTA is possible from a foreshortened apical two‐chamber view. The abdominal aorta is easily viewed from the subxiphoid window. BCA, brachiocephalic artery; LV, left ventricle; LCA, left carotid artery. With our current knowledge of the existence of Marfan‐like conditions such as LDS (see later), it is important to note that some patients with LDS may have been included in older reports on MFS. In particular, patients with widespread vascular involvement should be carefully reconsidered.
CHAPTER 33
Connective Tissue Disorders
Definition and pathophysiology of connective tissue disorders with cardiovascular involvement
Connective tissue
Connective tissue in the cardiovascular system
Angiotensin receptor blockade in Marfan syndrome
Recent concepts of the pathophysiology of genetic aortic disease
Heritable thoracic aortic disease and overview of the most relevant clinical entities with their underlying genetic defect
Imaging in connective tissue disorders
Marfan syndrome
Gene name
Syndrome name (if applicable)
Main cardiovascular features
Additional clinical features
HTAD related to genes encoding components of the extracellular matrix
FBN1
Marfan syndrome (MFS)
Sinus of Valsalva aneurysm, aortic dissection, mitral valve prolapse, main pulmonary artery dilation, left ventricular dilation and dysfunction
Lens subluxation, skeletal features (arachnodactyly, pectus deformity, scoliosis, flat feet, increased arm span, dolichocephaly), dural ectasia, striae
COL3A1
Vascular Ehlers–Danlos syndrome
Arterial rupture and dissection without preceding dilation/aneurysm
Gastrointestinal rupture, thin and translucent skin, dystrophic scars, facial characteristics (Madonna face, thin lips, deep‐set eyes), club feet, uterine rupture
LOX
Fusiform aneurysm of aortic root and ascending aorta
Scoliosis, joint hypermobility, skin striae
MFAP5
Aortic root aneurysm [44]
MFS features
BGN
Meester–Loeys syndrome [45] (autosomal recessive inheritance)
Early‐onset ascending aortic aneurysm and dissection
Facial dysmorphism, pectus deformities, joint hypermobility or contractures, skin striae
LTBP3
DASS (dental abnormalities and short stature) [46]
Aneurysms and dissections of the thoracic and abdominal aorta and other arteries
Dental abnormalities and short stature in homozygous/compound heterozygous state; dental abnormalities in heterozygous state
HTAD related to genes encoding components of the TGF‐β pathway
TGFBR1
Loeys–Dietz syndrome
Sinus of Valsalva aneurysm, aortic dissection, arterial aneurysms and dissections, arterial tortuosity, patent ductus arteriosus, atrial septal defect, bicuspid aortic valve
Bifid uvula/cleft palate, hypertelorism, pectus abnormalities, scoliosis, club feet
TGFBR2
SMAD2
TGFβ2
TGFβ3
SMAD3
Aneurysms osteoarthritis syndrome
Sinus of Valsalva aneurysm, aortic dissection, arterial aneurysms and dissections, arterial tortuosity
Osteoarthritis
HTAD related to genes encoding proteins involved in the contractile apparatus of vascular smooth muscle cells
ACTA2
Multisystemic smooth muscle dysfunction syndrome
Ascending aortic aneurysm, aortic dissection, patent ductus arteriosus, aortic coarctation, aortopulmonary window, pulmonary arterial hypertension
Congenital mydriasis, malrotation of the gut, moya‐moya like disease, periventricular white matter hyperintensities
FLNA
Ascending aortic aneurysm, aortic dissection, aortic regurgitation, patent ductus arteriosus, pulmonary arterial hypertension, mitral valve regurgitation
Periventricular nodular heterotopia (PVNH), joint hypermobility, soft skin, progressive obstructive lung disease [47]
MYLK
Mild ascending aortic dilation, aortic dissection with minimal aortic dilation
PRKG1
Sinus of Valsalva and ascending aortic aneurysm, aortic dissection
MYH11
Sinus of Valsalva and ascending aortic aneurysm, arterial aneurysm and dissection
LMOD1
Aortic aneurysm/dissection
Mild Marfanoid features [48]
Imaging
Imaging modality
Possible findings
Echocardiography
Aortic root dilation/dissection
Aortic valve morphology ± regurgitation
Ascending aortic dilation/dissection
Aortic arch dilation/dissection
Descending aortic dilation/dissection
Dilation of the proximal brachiocephalic vessels
Aortic arch tortuosity (in children)
Main pulmonary artery dilation
Mitral valve prolapse ± regurgitation
Tricuspid valve prolapse ± regurgitation
Left ventricular dilation
Left ventricular dysfunction
Coronary artery dilation (most common postoperatively)
CMR/CTA
Cardiovascular:
Aortic root dilation/dissection
Aortic valve morphology ± regurgitation (MRI)
Ascending aortic dilation/dissection
Aortic arch dilation/dissection
Descending aortic dilation/dissection
Dilation of brachiocephalic vessels
Aortic arch tortuosity
Main pulmonary artery dilation
Mitral valve prolapse ± mitral regurgitation (quantified by MRI)
Tricuspid valve prolapse ± tricuspid regurgitation (quantified by MRI)
Mild ventricular dilation (MRI)
Mild ventricular dysfunction (MRI)
Coronary artery dilation (most common postoperatively)
Arterial tortuosity (most evidence in vertebral arteries)
Small and medium artery aneurysm
Noncardiovascular:
Scoliosis/kyphosis/lordosis
Pulmonary emphysema/blebs (CT)
Diaphragmatic herniation/eventration
Dilation of the aortic root and ascending aorta
Dissection of the ascending aorta
Mitral valve prolapse
Dilation and dissection of the descending aorta
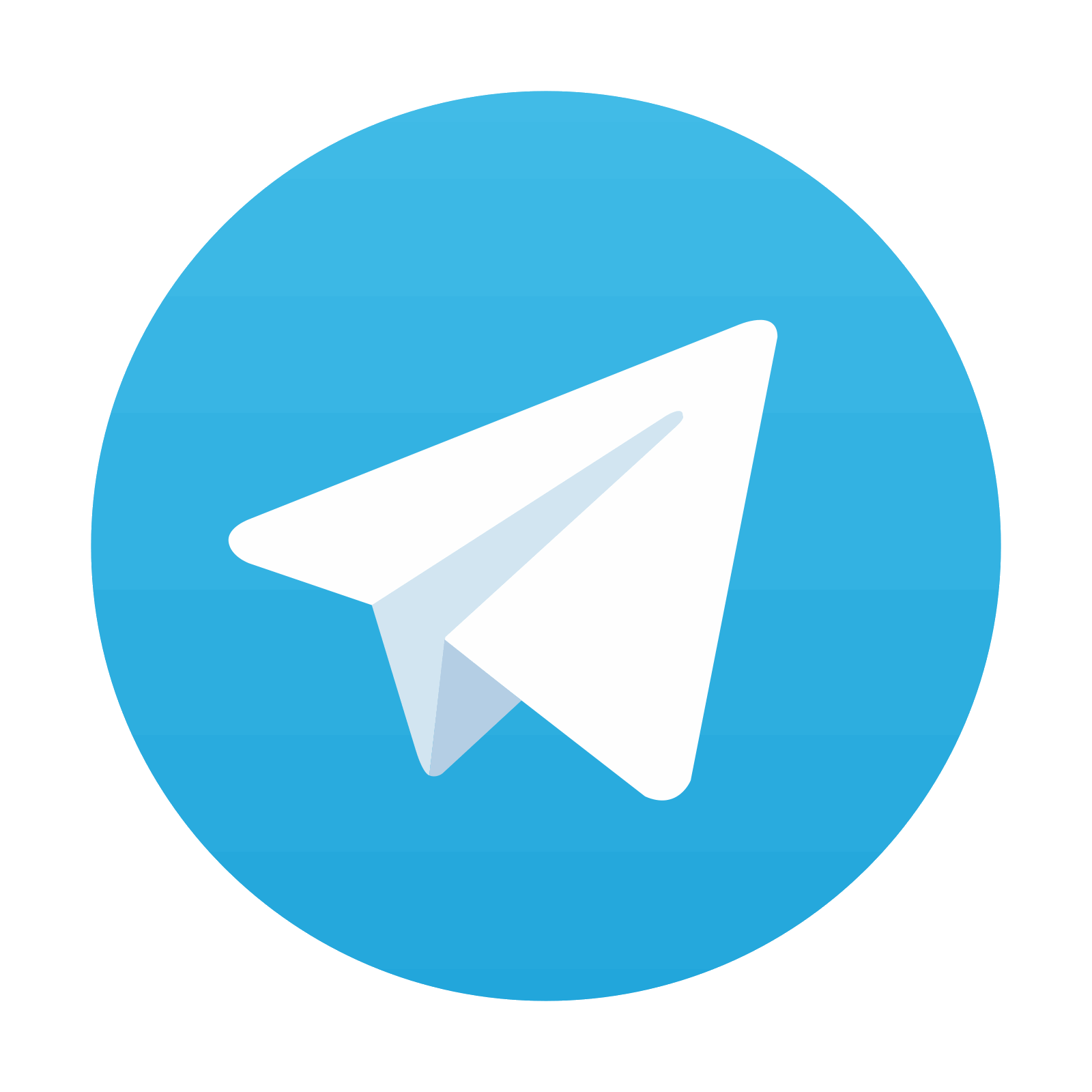
Stay updated, free articles. Join our Telegram channel
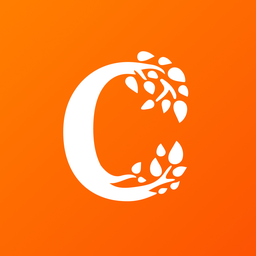
Full access? Get Clinical Tree
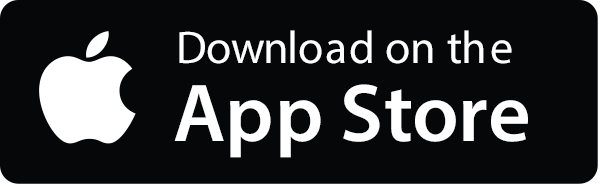
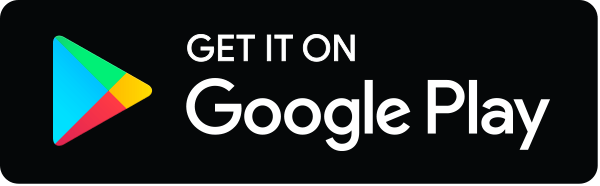