Shubhika Srivastava1 and Piers C. A. Barker2 1 Nemours/Alfred I. duPont Hospital for Children, Wilmington, DE, USA 2 Duke University School of Medicine, Durham, NC, USA The term coronary derives from the Latin root coronarius, which means crown. When viewed from the base, the right and circumflex coronary arteries can be viewed as forming a “crown” around the ventricles. Coronary artery anomalies, with and without concomitant structural congenital heart disease, are an important topic for the pediatric cardiologist. The spectrum of abnormalities is wide and the clinical presentation varies, both in terms of age (neonate to adult) and life events (rest, exercise, or pregnancy) [1,2]. This chapter reviews the imaging of coronary artery anomalies of importance to the pediatric cardiologist with a focus on echocardiography [3]. Further details about coronary artery anomalies associated with specific congenital heart defects are found in the respective chapters. Embryologic development of the coronary arteries extends from the endocardial sinuses to the epicardial network. Before the development of the coronary arteries, blood flow to the loosely packed myocardium of the embryonic heart is provided by sinusoids directly from the ventricular cavities. As the myocardium becomes more compact, veins and arteries develop within the epicardial layer, with the intramyocardial circulation developing within the myocardium itself. This parallel development of a compacted myocardium and the epicardial coronary artery system appears to be evolutionarily preserved in different species. In particular, a more compacted myocardium and more fully developed epicardial coronary artery system is seen in animals with greater aerobic cardiac demands, while more sedentary animals appear to maintain a noncompacted myocardium with greater sinusoidal perfusion [4,5]. An area of recent focus is the precise mechanisms of coronary angiogenesis, and how the immature coronary endothelial plexus, covered in pericytes, transforms from small vessels into mature, large‐diameter arteries coated in vascular smooth muscle that connect to the aorta. This endothelial plexus starts as a highly branched network of small, similarly sized vessels, and undergoes branching morphogenesis and massive expansion to cover and infiltrate the entire myocardium. Initially devoid of blood flow, the plexus eventually anastomoses with the aorta, which provides a source of arterial blood. This concept of ingrowth provides a rational basis for understanding the myriad patterns taken by anomalous coronary arteries, and is supported by the work of Bogers et al. [6] demonstrating that the arterial sprouts grow into the developing aortic sinuses rather than grow out from the arterial roots. More recently, defects in Tbx1 gene expression and development of the second heart field have been proposed as important to coronary artery patterning [7]. The development of the definitive coronary circulation begins at about 32 days’ gestation and is complete by about 45 days’ gestation. Coronary artery developmental abnormalities may also manifest in genetic syndromes, suggesting that the abnormalities evolve beyond early embryologic formation as a separate process from acquired atherosclerotic or inflammatory disease. Examples of these abnormalities include diffuse coronary artery stenosis, ostial stenosis, or coronary artery dilation seen in 45% of patients with Williams syndrome along with supravalvar aortic stenosis, or the coronary artery ectasia and aneurysms seen in Noonan and Marfan syndromes [8–10]. There are normally two major coronary arteries, which originate from aortic sinuses that “face” the pulmonary artery – the right and left aortic sinuses of Valsalva. The third, or posterior, sinus of Valsalva is the “nonfacing” sinus and is usually devoid of a coronary artery orifice. It is therefore termed the noncoronary sinus of Valsalva. The sinuses are not named after the coronaries originating from them, but rather after the ventricle they supply. For example, in an L‐looped heart the right coronary artery that supplies the L‐looped right ventricle (RV) would normally arise from the left sinus of Valsalva. The left main coronary artery (LMCA) arises from the left aortic sinus of Valsalva and courses only a short distance (<4 cm in the adult) before dividing into the left circumflex (LCx) and left anterior descending (LAD) coronary arteries (Figure 31.1). The LAD is classified into three types based on the length of the septum it supplies [11]. The LCx varies considerably in size depending on whether the posterior descending coronary artery is a branch of the right coronary artery (RCA) (right‐dominant coronary system) or a branch of the LCx (left‐dominant coronary system). Rarely a co‐dominant coronary circulation may be present in which both the right and circumflex coronary arteries contribute to the posterior descending coronary artery. If the posterior descending coronary artery originates from the LCx (in about 10% of patients), the LCx is large. In some patients with a dominant RCA, the LCx may be small. Figure 31.1 The normal left coronary artery system. LAD, left anterior descending coronary arteries; LCx, left circumflex; LM, left main coronary artery. The LCx travels under the left atrial appendage and along the left atrioventricular (AV) groove. In 40% of cases, the sinus node artery arises from the LCx. The sinus node artery courses under the left atrial appendage, penetrates the interatrial septum, encircles the base of the superior vena cava, and supplies the sinus node. When the sinus node artery is not a branch of the LCx, it is a branch of the RCA. Kugel’s artery arises from the LCx, RCA, or LMCA and courses through the interatrial septum, anastomosing with the artery to the AV node. Obtuse marginal branches arise from the LCx and supply areas of the lateral wall of the left ventricle (LV). The left atrial circumflex artery may arise from the LCx and supply the left posterior atrial wall. Occasionally, the sinus node artery can arise from the left atrial circumflex coronary artery. The LAD appears to be a direct continuation of the LMCA rather than a branch of it. The LAD travels along the interventricular sulcus and may continue around the apex of the heart onto the posterior interventricular sulcus to anastomose with the posterior descending coronary artery. The left conus artery is the first branch of the LAD and may anastomose with the conal branch of the RCA, forming the circle of Vieussens. Approximately four to six septal branches of the LAD penetrate the ventricular septum and anastomose with posterior septal branches arising from the posterior descending coronary artery. The LAD gives rise to diagonal branches that supply the anterior surface of the ventricles and the right ventricular infundibulum. A common variation is for the LMCA to trifurcate into LAD, LCx, and so‐called ramus intermedius branches [12]. Most important for pediatric and congenital echoradiographers is not to presume the ramus intermedius branch to be either the LAD or LCx, thus leading to a potentially incomplete or inaccurate diagnosis of normal coronary artery anatomy. Dual LADs have been reported in adult radiological studies with an incidence varying from 1.3 to 4%. This branching pattern variant is benign but has implications for the assessment of coronary atherosclerosis in adults. In addition, there are multiple other subtypes of LAD anatomy, which are defined based on the territory supplied and aortic sinus of origin, including origins from the appropriate sinus, wrong sinus, or even the RCA [13]. The RCA arises from the right aortic sinus of Valsalva and courses behind the main pulmonary artery, beneath the right atrial appendage, and along the right AV groove (Figure 31.2). The RCA branches at the acute margin of the heart (lateral aspect of the right AV groove) to give rise to the acute marginal coronary artery, which courses posteriorly and caudally over the diaphragmatic surface of the heart and forms the largest branch supplying the RV free wall. Other branches of the RCA include the right conal branch, the sinus node artery, an atrial branch, RV muscle branches (additional acute marginal branches), AV node artery, the posterior descending coronary artery, and septal branches. The right conus artery is the first branch of the RCA in about 50% of cases; in the others, it arises directly from the right aortic sinus of Valsalva through a separate ostium (normal variant). The sinus node artery arises from the RCA in 60% of cases and from the LCx in the remainder. Figure 31.2 The normal right coronary artery system. AV, atrioventricular; SA, sinoatrial. Around 90% of the population has a dominant RCA system, that is, the posterior descending coronary artery is a branch of the RCA. In the remainder, the posterior descending coronary artery originates from the LCx. The term “dominant RCA system” refers only to the origin of the posterior descending coronary artery, not to the coronary artery that supplies most of the myocardium. Indeed, except in unusual circumstances, the majority of the myocardium is supplied by the LCA regardless of the “dominance” of the coronary artery system. The posterior descending coronary artery supplies the lower portion of the ventricular septum. The septal branches of the posterior descending coronary artery anastomose with the septal branches of the LAD. Muscular branches supplying the diaphragmatic portions of the right and left ventricles also arise from the posterior descending coronary artery. The artery to the AV node originates from the posterior descending coronary artery at or near the crux of the heart. The coronary arteries provide a flow of oxygenated blood to the almost exclusively aerobic myocardium, and a delicate balance is maintained between myocardial oxygen supply and demand (Figure 31.3). Upsetting this balance produces myocardial ischemia and thus impaired ventricular contraction. The three primary determinants of myocardial oxygen demand are systolic wall tension, contractility, and heart rate. Pressure work increases myocardial oxygen consumption to a greater extent than does volume work. Myocardial oxygen supply is determined by the oxygen content of the blood and by coronary blood flow. Coronary blood flow is in turn determined by coronary perfusion pressure and by coronary vascular resistance. Figure 31.3 The delicate balance between myocardial oxygen supply and demand and the factors that influence this balance. Source: Adapted from Ardehali A, Ports TA. Myocardial oxygen supply and demand. Chest 1990;98:699–705. The American College of Chest Physicians. Coronary perfusion pressure equals coronary artery pressure minus downstream pressure and is different in various regions of the myocardium. In the absence of a fixed or dynamic obstruction in the coronary circulation, coronary artery pressure equals aortic pressure. Distal to such an obstruction, coronary artery pressure and coronary perfusion pressure are reduced. Coronary vascular resistance is influenced by metabolic, humoral, and neural factors, and also by autoregulation and by extravascular compressive forces. The importance of extravascular compressive forces as determinants of vascular resistance is unique to the myocardium. Myocardial wall tension is so great in the LV during systole that sufficient extrinsic compression is placed on the coronary arteries to cause coronary vascular resistance to approach infinity and coronary blood flow to approach zero. Thus, the vast preponderance of coronary blood flow to the LV occurs during diastole. Compressive forces exerted by the RV are ordinarily far lower than those of the LV; therefore, coronary blood flow to the RV is not interrupted during ventricular systole. However, such flow interruption may occur with RV hypertension [14]. Normally, coronary blood flow far exceeds the metabolic demands of the myocardium, allowing for a large safety margin to protect the heart from ischemia. This concept has been expressed as coronary flow reserve, which is the difference between baseline and maximal coronary blood flow at any given perfusion pressure. When coronary flow reserve is absent, myocardial ischemia ensues. Coronary flow reserve may decrease if baseline coronary blood flow increases or if maximal coronary blood flow decreases. Causes of an increase in baseline coronary blood flow include exercise, anemia, hypoxemia, tachycardia, thyrotoxicosis, and ventricular hypertrophy. Causes of a decrease in maximal coronary blood flow include stenosis or occlusion of the large or small coronary arteries, an increase in LV pressure without a corresponding increase in perfusion pressure, tachycardia, and polycythemia [14,15]. The subendocardium is particularly susceptible to ischemia. This is due to this region’s limited reserve for vasodilation, increased extrinsic compression from the higher wall stress to which it is subjected, and its resultant higher metabolic demands. This region is therefore often the first to manifest ischemic changes. Assessment of regional function and wall motion abnormalities has become increasingly important in pediatric imaging given the increased detection and interventions for coronary artery anomalies, the increase in coronary translocation procedures accompanying repair of other defects, and the persistence of inflammatory states such as Kawasaki disease. The 17‐segment American Heart Association model has been shown to have best agreement with the available anatomic data and has the best fit with the methods commonly used in both echocardiography and single‐photon emission computed tomography (SPECT) nuclear cardiology [16]. For the model, the anterolateral papillary muscle blood supply is considered to come from the LAD and the diagonal or a marginal branch of the LCx. The LCx or RCA (depending on dominance) provides the blood supply to the posteromedial papillary muscle. Two‐dimensional (2D) echocardiography with color Doppler is an established tool in the diagnosis and functional evaluation of infants and children with suspected anomalies of the coronary arteries [3]. The excellent temporal and spatial resolution of 2D echocardiography allows for good diagnostic accuracy of imaging the origins and proximal course of the coronary arteries in general and, in particular, in small patients with high heart rates. Both spatial and temporal resolution vary with probe choice, patient habitus, and instrument settings, and quality of echocardiographic images is fundamentally dependent on operator skill. Nevertheless, in skilled hands, both transthoracic (TTE) and transesophageal echocardiography (TEE) can reliably image the proximal coronary arteries; mid and distal coronary artery branches are typically not well delineated. Although TEE imaging of the coronary arteries is seldom performed as the initial diagnostic study in the pediatric population, it is important to include a careful assessment of the coronary artery anatomy as part of a complete intraoperative study, given the association of coronary anomalies with different congenital heart defects, the dramatic consequences that a new coronary diagnosis can have on the operative plan, and the potential to avoid serious morbidity [17–19]. Given that missing a coronary anomaly can have major adverse clinical implications, every initial echocardiographic examination should include detailed evaluation of the origins and proximal courses of the coronary arteries [3,20]. Optimizing instrument setting to obtain the best signal‐to‐noise and contrast‐to‐noise ratio is paramount to ensure high‐quality imaging of the coronary arteries. The highest‐frequency probe that allows adequate penetration should be used. Often this will be a higher‐frequency probe than would typically be used for a particular view, taking advantage of the superficial epicardial location of the proximal coronary arteries being in the near‐field of the sector. The proximal coronary arteries are best imaged in the parasternal long‐ and short‐axis planes, and, in infants and young children, from the subxiphoid coronal (long‐axis) view. These views combined will demonstrate the origins and proximal courses of the coronary arteries in terms of both horizontal and vertical locations relative to the aortic root. In the parasternal short‐axis view at the base of the heart, both right and left main coronary artery origins – often imaged simultaneously – may be seen to arise from their respective sinuses of Valsalva (Figure 31.4, Video 31.1). Clockwise rotation of the probe is often necessary for optimal imaging of the LMCA and its bifurcation into LAD and LCx branches (Figure 31.5, Video 31.2). Minor adjustments are often needed from the LMCA bifurcation view to visualize the LAD in greater length with and without color Doppler to exclude confusion with the edge of the left atrial appendage or fluid in the left anterior AV sulcus (Figure 31.6, Video 31.3). Either clockwise or counterclockwise rotation may be necessary for optimal imaging of the RCA (Figure 31.7, Video 31.4 and 31.5). These movements are very subtle. Figure 31.4 Parasternal short‐axis image at the base of the heart showing normal origins of the right and left coronary arteries. The left main coronary artery (LMCA) is seen arising from the aortic left sinus of Valsalva with extension into the left anterior descending coronary artery. The right coronary artery (RCA) is seen arising from the right aortic sinus of Valsalva with a proximal course in the anterior right atrioventricular groove. The asterisk (*) marks the position of the intercoronary commissure of the aortic valve. Figure 31.5 Parasternal short‐axis image at the base of the heart demonstrating the normal bifurcation of the left main coronary artery into left anterior descending (LAD) and left circumflex (LCx) branches. Use of color Doppler confirms normal prograde flow in each proximal coronary artery segment. Ao, aorta. Figure 31.6 Parasternal short‐axis image at the base of the heart demonstrating the left anterior descending (LAD) artery in greater length. Use of color Doppler excludes confusion of the 2D image with the edge of the left atrial appendage or fluid in the left anterior atrioventricular sulcus. Ao, aorta. Figure 31.7 Parasternal short‐axis image dedicated to the origin and proximal course of the right coronary artery (RCA), with confirmation of normal prograde flow by color Doppler. Ao, aorta. One common practice that should be stringently avoided when imaging coronary arteries is that of obtaining still frames of the arterial origins and making diagnostic decisions based upon these images. With the anomalous aortic origin of a coronary artery from the pulmonary trunk or the aorta, the proximal coronary artery often approaches the appropriate sinus of Valsalva, and resolving the thin tissue between the coronary arterial and aortic lumens can be difficult or misleading based on a still frame. It is easy, therefore, to be falsely assured of a normal origin of the coronary artery when, in fact, the coronary artery arises anomalously from another location. From the parasternal long‐axis view, leftward angulation of the probe allows visualization of the bifurcation of the LMCA and a long section of the LAD as it courses along the anterior interventricular septum (Figure 31.8, Video 31.6). From the subxiphoid coronal (long‐axis) view at the level of the aortic root, the proximal left and right coronary arteries may be visualized relative to the sinotubular junction (Figure 31.9, Video 31.7). Figure 31.8 Parasternal long‐axis image with leftward angulation to demonstrate the bifurcation of the left main coronary artery (LMCA) and a long section of the left anterior descending (LAD) coronary artery along its course in the anterior interventricular groove. LCx, left circumflex coronary artery. Source: Image courtesy of Dr. Greg Ensing, University of Michigan. Figure 31.9 Subxiphoid coronal (long‐axis) image demonstrating the origins of the right and left coronary arteries relative to the aortic sinotubular junction. Ao, aorta; LMCA, left main coronary artery; LV, left ventricle; RCA, right coronary artery; RV, right ventricle. Although more technically difficult, the distal portions of the coronary arteries may be visualized from several views. The distal RCA may be seen in the posterior right AV groove from an apical four‐chamber view (Video 31.8), whereas the distal LCx may be seen in the same view in the posterior left AV groove. The posterior descending coronary artery is often best seen from inferiorly angled parasternal long‐axis or posteriorly tilted apical four‐chamber views (Figure 31.10, Video 31.9). Figure 31.10 Apical four‐chamber view with posterior tilt demonstrating the distal course of the posterior descending coronary artery (PDA). Source: Image courtesy of Dr. Greg Ensing, University of Michigan. In addition to this anatomic information, echocardiography may provide important functional information about the coronary arteries. The direction of flow in the coronary arteries is demonstrated by color Doppler; this may provide critical information regarding anomalies of coronary artery origin from the pulmonary artery, as described later. Color Doppler imaging of either the right or left coronary artery origin from the parasternal short‐axis view may be challenging due to the perpendicular position of the artery relative to the angle of insonation; great care should therefore be taken to unambiguously visualize antegrade flow from the aortic root into the proximal coronary artery to confirm a normal origin. Careful attention to direction of color flow is also critical to avoid confusion between coronary artery and coronary vein flow, especially when visualizing distal segments. Pulsed‐wave Doppler interrogation is possible, although more easily performed by transesophageal imaging, and normal but different flow patterns have been described for both the right and left coronary arteries as expected based on the physiology. Specifically, flow in the RCA is seen during both diastole and systole, while left coronary flow is predominantly in diastole [21,22]. Both systolic and diastolic function can be altered in certain forms of congenital coronary artery anomalies and these parameters may be readily evaluated with echocardiography. Chapter 7 reviews echocardiographic assessment of global and regional left ventricular systolic function, Chapter 8 discusses evaluation of diastolic function, and Chapter 9 describes methods for assessment of right ventricular function. The coronary arteries are rarely imaged well by fetal echocardiography. Exceptions to this rule include congenital coronary artery anomalies that lead to significant dilation of the coronary arteries and/or abnormal flow from the coronary arteries into a cardiac chamber or great vessel. Specific examples include coronary–cameral fistulae, and either pulmonary atresia with intact ventricular septum or hypoplastic left heart syndrome with sinusoidal connections to the ventricular myocardium [423–26]. Occasionally a very large fistula can result in significant diastolic steal from the fetal cerebral circulation. In fetal life, myocardial lactate oxidation constitutes the major energy source. The high oxygen extraction (70−80%) and arteriovenous oxygen difference (14 mL/dL) in the resting state allow for little increase in oxygen delivery when cardiac demand increases. Such increased oxygen demand is almost exclusively met by augmentation of coronary blood flow through autoregulation and the difference between the aortic diastolic pressure and right atrial pressure as the driving forces for coronary perfusion [27]. Prominent coronary flow visualization on routine color Doppler is often noted on fetal echo in pregnancies complicated by fetal growth restriction, fetal anemia, or ductus arteriosus constriction. This finding, along with absent or reduced diastolic flow in the umbilical artery, coincides with worsening fetal outcomes [28,29]. Cardiac catheterization with aortic root angiography or, preferably, selective coronary arteriography, has long been the gold standard in the diagnosis of congenital and acquired coronary artery anomalies. However, with major technological advances in echocardiography, cardiac magnetic resonance (CMR) imaging, and cardiac computed tomography (CCT), many congenital and acquired coronary anomalies in infants and children can now be evaluated noninvasively [30–32]. With evolving techniques to minimize radiation exposure and improved imaging sequences, use of CCT and CMR is rapidly expanding into the pediatric population [33–35]. Chapter 47 reviews the technical aspects and clinical applications of multimodality imaging, including evaluation of coronary anomalies. Although catheterization remains the gold standard for detection of distal coronary artery disease, CCT is superior for detection of anomalies of coronary artery origin or proximal course [36]. More recently, angiography has been combined with intravascular ultrasound (IVUS), direct measurements of fractional flow reserve, or optical coherence tomography. While these additional procedures are rarely used in children, there are a few reports of their use in children to aid the evaluation of Kawasaki disease, anomalous aortic origins of the coronary arteries, and myocardial bridges [37,38]. With recent technological advances, increased use of beta blockade for heart rate control and nitroglycerin for coronary vasodilation, and refinement of imaging protocols in infants and children, the role of CCT in evaluation of the coronary arteries in the pediatric age group continues to increase [30,33,35]. Several invasive techniques for assessing fractional coronary flow reserve exist, including perfusion imaging and calculation of the transluminal attenuation gradient. Noninvasive assessment of fractional flow reserve by CT has received substantial attention, although its use is still far from universal. Calculated as the ratio between the maximal coronary flow across a stenosis and the maximal coronary flow without stenosis under hyperemic conditions (beta blockade plus nitroglycerin or adenosine), a positive result is 0.75 (meaning a 25% reduction in flow due to a stenosis). The technique has demonstrated significant incremental benefit with excellent sensitivity and specificity for the diagnosis of clinically important coronary artery stenosis in adults [39,40]. Use of this technique in adults has been limited by cost, data transfer for offsite calculation until recently, and limited vendors providing this service. Its use in pediatrics or for congenital coronary artery abnormalities has remained limited. Standard nuclear stress perfusion imaging (e.g., single‐photon emission computed tomography or SPECT) is a modality rarely used in children due to relatively low sensitivity and specificity, and the risks of increased radiation exposure [3]. However, there is growing expertise with the use of positron emission tomography (PET)‐CT for assessment of myocardial perfusion and viability in older children and young adults with congenital and acquired coronary anomalies (see Chapter 47) [41]. CMR allows radiation‐free evaluation of myocardial perfusion and viability in infants and children. The stress component is performed using adenosine or a derivative to produce coronary artery vasodilation, followed by injection of a gadolinium‐based contrast agent. Contrast will then preferentially perfuse myocardial segments that are vasodilated, producing increased contrast distribution, with proportionally less perfusion to segments supplied by a stenotic coronary artery. Exercise using supine cycle ergometry in patients old enough to cooperate and dobutamine stress in younger patients have also been used [42]. These stress MRI techniques have been demonstrated to be safe and both highly sensitive and specific for the detection of coronary artery disease in at‐risk pediatric patients [43–45]. Beyond the assessment of differences in regional myocardial perfusion, cardiac MRI also permits the distinction between inducible ischemia and infarction through the use of delayed enhancement imaging performed after the administration of gadolinium (see Chapter 47). Regions of the heart with increased extracellular space (e.g., replacement fibrosis) will retain gadolinium longer than viable myocardium, and thus appear hyperenhanced compared to normal myocardium. On stress imaging, therefore, an ischemic yet viable region will have decreased perfusion with adenosine but no corresponding fibrosis with delayed enhancement, while an infarcted region will have both decreased perfusion and matching delayed enhancement. Somewhat counterintuitively, rest imaging is most helpful to determine if a perfusion defect at stress represents a true perfusion abnormality or an artifact. This technique has been shown to be superior to others for the detection of coronary ischemia due to coronary artery disease in adults [46] and its use is increasing in children [44,45]. The recent development of black‐blood delayed enhancement sequences that better demonstrate subendocardial scar adds further to the diagnostic strength of this modality [47]. A classification of congenital coronary artery anomalies is provided in Table 31.1. Other classifications have been published [48–51]. Table 31.1 Classification of congenital coronary artery anomalies Minor variations in coronary artery anatomy are those that are hemodynamically insignificant and not associated with an increased risk of sudden death [52]. Their presence must nonetheless be recognized in order to interpret coronary arteriograms and echocardiograms properly, to avoid inadequate myocardial protection when cardioplegia is given selectively into the coronary orifices, and to avoid coronary injury at the time of surgery. Absent LMCA with separate origin of the LAD and LCx from the left sinus of Valsalva has been reported in 0.4% of the population and is associated with left coronary dominance and myocardial bridging [53]. The other most common minor variation in coronary artery anatomy is the origin of the LCx from the right aortic sinus. After its aberrant origin, the artery courses posterior to the aorta to reach the left AV groove. This variant occurs in about 0.5% of individuals undergoing coronary arteriography and is compatible with normal life. The anatomy is most often recognized echocardiographically by seeing a horizontal linear lucency (the proximal aberrant circumflex coronary artery) coursing posterior to the aorta from the apical four‐chamber view (Figure 31.11). Origin of either the right or the left coronary artery from the posterior noncoronary sinus of Valsalva is exceedingly rare (0.003% to 0.0008%, respectively) and is generally considered a benign anomaly as long as the coronary ostium is normal and the proximal course is not intramural (Video 31.10 and 31.11) [36]. These variations are important to recognize, especially when cardioplegia needs to be delivered if there are separate ostial origins. Figure 31.11 Apical four‐chamber view tilted slightly anteriorly (between the atrioventricular valves and aortic valve), demonstrating retro‐aortic course of the left circumflex coronary artery (LCx) originating from the right coronary artery (not seen). Variations in the number of coronary artery ostia have been described [53,54]. Some reports have suggested that in approximately 50% of cases the conus artery originates from a separate orifice in the right aortic sinus [55]. Less commonly, in approximately 1% of the general population, the LCx has a separate orifice in the left coronary sinus [48,53,56].Very rarely, these two anomalies occur together and produce four coronary ostia. Finally, variations in the position of the coronary artery ostia are also common. Normally, in adults, the coronary artery ostia are located just below the aortic sinotubular junction (STJ). However, ostial positions above or below this occur in up to 40% of individuals undergoing coronary arteriography. Such variations appear to be benign [49]. Anomalous origin of the LMCA from the pulmonary artery (ALCAPA) is one of the most important congenital coronary artery anomalies for the pediatric cardiologist since it represents the rare opportunity to successfully treat a form of dilated cardiomyopathy [57]. Pulmonary artery origin of coronary arteries was first described by Brooks in 1885 [58] and was further discussed by Abbott in 1908 [59]. The first clinical description of this anomaly, however, was given eloquently by Bland, White, and Garland in 1933 [60] and the ALCAPA syndrome often bears the eponym Bland–White–Garland syndrome. This rare anomaly occurs in approximately 1 per 300,000 live births and represents 0.5% of cases of congenital heart disease [61]. It generally occurs as an isolated anomaly but has been associated with patent ductus arteriosus, ventricular septal defects, AV canal defects, tetralogy of Fallot, coarctation of the aorta, hypoplastic left heart syndrome, and truncus arteriosus [52,62–64]. The LMCA usually originates from either the left or the posterior sinus of the pulmonary artery (Figure 31.12). It can also originate more distally and even from the undersurface of the proximal right pulmonary artery. It then branches and distributes in a manner analogous to a normally arising left coronary artery. It is therefore only the origin of the coronary artery that is abnormal. Figure 31.12 Parasternal short‐axis image demonstrating the left main coronary artery (LMCA) arising anomalously from the posterior sinus of the main pulmonary artery (MPA) with distal bifurcation into typical anterior descending and circumflex branches. Note the proximity of the anomalous LMCA origin to the intercoronary commissure of the aortic valve. Ao, aorta. The physiology of this anomaly depends on the blood flow in the left coronary artery (Figure 31.13). Classically, four hemodynamic phases have been described [65–67]. In the first phase, pulmonary vascular resistance and pulmonary arterial pressure are high and the anomalous coronary artery is supplied by blood from the pulmonary artery. This occurs during fetal life and in the newborn period and does not produce myocardial ischemia. The second phase is the transitional period during which pulmonary vascular resistance and pulmonary arterial pressure fall. During this time, flow into the left coronary artery reverses, that is, from aortic root to the RCA to the left coronary artery via collaterals. This phase typically occurs in the newborn and early infancy. Depending on the extent of the collateral circulation, this physiology can result in decreased perfusion pressure and flow to the vascular bed supplied by the LMCA and its branches, leading to myocardial ischemia. The third, or adult, phase is characterized by an extensive collateral circulation between the right and left coronary arteries, resulting in adequate perfusion of the myocardium that would normally be supplied by the left coronary artery. Blood flows from the RCA into the left coronary circulation and then into the pulmonary artery. There are no signs of myocardial ischemia in this phase. In the fourth phase, the left coronary artery no longer functions as a “feeding vessel” to the left ventricular myocardium but rather as a direct conduit from the RCA to the pulmonary artery. That is, there is “steal” of oxygenated blood from the arteriolar and capillary vessels of the left coronary circulation, resulting in myocardial ischemia. Figure 31.13 Diagrammatic portrayal of varying functional states in anomalous origin of the left coronary artery from the pulmonary trunk. (a) During fetal life aortic (A) and pulmonary arterial (P) pressures are essentially equal. Flow in the anomalous artery is from the pulmonary trunk into the myocardium. (b) In early postnatal life, pulmonary pressure falls and extensive intercoronary collateral channels have not yet developed. This results in decreased perfusion pressure and flow through the anomalous coronary artery, often leading to development of myocardial ischemia. (c) In the final phase, a rich collateral system has developed between the right and left coronary systems. Characteristics of an arteriovenous fistula now pertain, with the major contribution to fistulous flow coming from the right coronary artery. Mediastinal arteries, which make communication with the coronary arterial system, may also contribute to such flow. Source: Edwards JE. The direction of blood flow in coronary arteries arising from the pulmonary trunk. Circulation 1964;29:164. © 1964, Wolters Kluwer. Not all patients pass consecutively through each of these phases, and overlap can be present between different phases. Indeed, most patients present in infancy during the transitional phase and undergo surgical repair. A few patients have adequate collateral vessels between the right and left coronary arteries and escape diagnosis early in life, presenting in adulthood in phase 3 or 4. The schema nonetheless represents a useful framework for the description of the varied physiologies with which these patients may present. The majority of patients present in early infancy with failure to thrive, tachypnea, dyspnea, wheezing, diaphoresis, or angina‐like episodes. In infantile cases, there is a 2 : 1 male‐to‐female preponderance [17]. Classically, infantile angina is brought on by feeding, consists of “paroxysmal attacks of acute discomfort precipitated by the exertion of nursing,” and is sometimes also called “cardiac colic” [60]. Examination of such infants shows the signs of congestive heart failure. Either no systolic murmur or a very short grade 1–2 systolic murmur is heard. Occasionally, the murmur of mitral regurgitation may be the only presenting sign. The etiology of the mitral regurgitation is some combination of ischemia or infarction of the papillary muscles and annular dilation due to the ischemic dilated cardiomyopathy. Occasionally, patients remain asymptomatic during infancy. These are patients who have extensive collateral coronary arteries and therefore present in later childhood or adulthood with varying murmurs, sudden cardiac death, congestive heart failure, or incidental cardiomegaly or other abnormalities serendipitously revealed on chest x‐ray or ECG [19,68,69]. In one relatively large review of the adult experience with this lesion, the mean age at diagnosis was 41 years, with the oldest patient diagnosed at age 83 years. Most patients were symptomatic with congestive heart failure, ventricular arrhythmias, or sudden cardiac death, and almost all had an abnormal ECG [70]. Another group of patients who escape symptomatology in infancy are those with ALCAPA syndrome and a concomitant congenital or acquired heart disease that preserves or produces pulmonary hypertension [71–73], or patients with left main ostial stenosis limiting the coronary “steal.” These patients may present with severe LV dysfunction following repair of the associated lesion if the anomalous left coronary goes unrecognized. The typical echocardiographic findings are of a dilated, hypocontractile LV, a dilated left atrium, and moderate or greater mitral regurgitation (Video 31.12). There is eccentric left ventricular hypertrophy characterized by increased left ventricular mass index with normal or, more typically, reduced mass‐to‐volume ratio. The left ventricular dysfunction is global with regional wall motion abnormalities [74]. All of these findings may be seen with myocarditis and dilated cardiomyopathy in addition to the ALCAPA syndrome. Hence, the coronary arteries must be diligently evaluated in any patient who presents with these echocardiographic findings. Two‐dimensional imaging of the coronary arteries alone is inadequate to distinguish ALCAPA from other conditions. The RCA is often dilated in the ALCAPA syndrome [75], with one study reporting a RCA to aortic root ratio of greater than 0.2 highly specific for this diagnosis [19,76
CHAPTER 31
Congenital Anomalies of the Coronary Arteries
Definition
Developmental considerations
Normal coronary anatomy
Left coronary artery
Right coronary artery
Physiology
Imaging
Goals of the examination and transthoracic imaging
Functional imaging – wall motion abnormalities
Prenatal assessment
Imaging of the adult and multimodality imaging techniques
Assessment of fractional coronary flow reserve
Perfusion imaging
Congenital coronary artery anomalies
Minor variations in coronary artery anatomy
Anomalies of coronary arterial origin
Anomalous left main coronary artery from the pulmonary artery
Physiology
Clinical features
Imaging
Stay updated, free articles. Join our Telegram channel
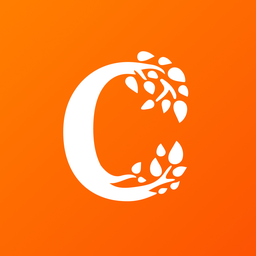
Full access? Get Clinical Tree
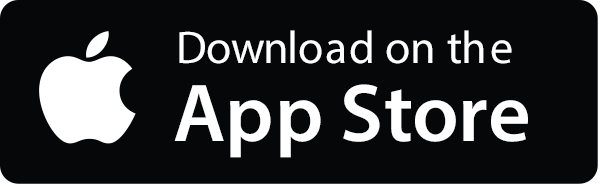
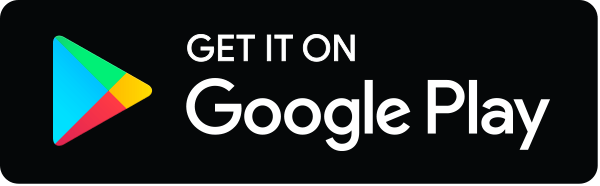