Jan D’hooge1, Olivier Villemain2, and Luc L. Mertens2 1 Catholic University of Leuven; University Hospitals Leuven, Leuven, Belgium 2 The Hospital for Sick Children; University of Toronto, Toronto, ON, Canada In echocardiography images of the cardiovascular structures are created by using ultrasound waves. Knowledge of the physics of ultrasound helps us to understand how the different ultrasound imaging modalities operate and is also important when operating an ultrasound machine as it can help with image optimization during acquisition and a better understanding of machine operation. This chapter describes the essential concepts of how ultrasound waves can be used to generate an image of the heart. Certain technological developments are discussed, as well as how machine settings influence image characteristics. For a more detailed description of ultrasound physics in imaging we refer the readers to dedicated literature on the topic [1,2]. To illustrate how ultrasound imaging works, the acoustic “pulse–echo” experiment can be used: Figure 1.1 Local tissue compression due to deformation of the piezoelectric crystal (C) when applying an electric field. In the example shown in Figure 1.3, taken from a water tank experiment, two strong specular reflections can be observed (around 50 and 82 μs) while the lower amplitude reflections in between are scatter reflections. In clinical echocardiography, the most obvious specular reflection is the strong reflection coming from the pericardium observed in the parasternal views as a consequence of the high acoustic impedance difference. The direction of propagation of the specular reflection is determined by the angle between the incident wave and the reflecting surface. Thus, the strength of the observed reflection will depend on: (i) the exact transducer position; (ii) orientation with respect to the pericardium; and (iii) the acoustic impedance difference between the two structures. Indeed, for given transducer positions/orientations, the strong specular reflection might propagate in a direction not detectable by the transducer. For this reason, the pericardium typically does not show as bright in the images taken from an apical transducer position. In contrast, scatter reflections are not angle dependent and will always be visible for a given structure independent of the exact transducer position. Figure 1.2 The local tissue compression/decompression propagates away from its source at a speed of approximately 1530 m/s in soft tissue. Figure 1.3 The reflected amplitude of the reflected ultrasound waves as a function of time after transmission of the ultrasound pulse is called the radiofrequency signal. The total duration of the above described pulse–echo experiment is about 100 μs when imaging at 5 MHz The reflected signal in Figure 1.3 is referred to as an A‐mode image (“A” referring to “amplitude”) and is the most fundamental form of imaging given it tells us something about the acoustic characteristics of the materials in front of the transducer. For example, Figure 1.3 clearly shows that at distance of ~3.7 cm in front of the transducer the propagation medium changes density and/or compressibility, with a similar change occurring at a distance of ~6.3 cm (these distances correspond to 50 and 82 μs, respectively, times 1530 m/s – which is the total propagation distance of the wave – divided by 2 as the wave has to travel back and forth). The 2.6 cm of material in between these strong reflections is acoustically inhomogeneous (i.e., shows scatter reflections) and thus contains local (very small) fluctuations in mass density and/or compressibility, while the regions closer and further away from the transducer do not cause significant scatter and would thus be acoustically homogeneous. Indeed, this A‐mode image was taken from a 2.6‐cm thick tissue‐mimicking material (i.e., gelatin in which small graphite particles were dissolved) put in a water tank. Since the A‐mode image just presented is not visually attractive, the RF signal resulting from a pulse–echo experiment is further processed: Figure 1.4 The radiofrequency signal is demodulated in order to detect its envelope. This envelope signal (bold) is color encoded based on the local signal amplitude. Figure 1.5 The logarithmic compression curve. Figure 1.6 Translation of the ultrasound source results in a linear image format (a) whereas pivoting results in sector images (b). In order to obtain an ultrasound image, the procedures of signal acquisition and post‐processing are repeated. For conventional B‐mode imaging (“B” referring to “brightness”), the transducer can either be translated (Figure 1.6a) or tilted (Figure 1.6b) within a plane between two subsequent pulse–echo experiments. In this way, a conventional 2D cross‐sectional image is constructed. The same principle can be used for 3D imaging by moving the ultrasound beam in 3D space between subsequent acquisitions. Alternatively, the ultrasound beam is transmitted in the same direction for each transmitted pulse. In that case, an image line is obtained as a function of time, which is particularly useful when looking at motion. This modality is therefore referred to as M‐mode (“M” referring to “motion”) imaging. In the construction of an ultrasound image by focused waves, the assumption is made that all reflections originate from a region directly in front of the transducer. Although most of the ultrasound energy is indeed centered on an axis in front of the transducer, in practice part of the energy is also directed sideways (i.e., directed off‐axis). The former part of the ultrasound beam is called the main lobe whereas the latter is referred to as the side lobes (Figure 1.7, Videos 1.1 and 1.2). Because the reflections originating from these side lobes are much smaller in amplitude than the ones coming from the main lobe, they can typically be neglected. However, image artifacts can arise when the main lobe is in an anechoic region (e.g., a cyst or inside the left ventricular cavity) causing the relative contribution of the side lobes to become significant. In this way, a small cyst or lesion may be more difficult to detect, as it appears brighter due to spillover of (side lobe) energy from neighboring regions. Similarly, when using contrast agents, the reflections resulting from small side lobes may become more significant as these agents strongly reflect ultrasound energy. As such, increased brightness may appear in regions adjacent to regions filled with contrast without contrast being present in the region. Figure 1.7 Reflections caused by side lobes (red) will induce image artifacts because all reflections are assumed to arrive from the main ultrasound lobe (green). When the reflected wave arrives at the transducer, part of the energy is converted to electric energy as described in the previous section. However, due to the acoustic impedance difference between the tissue and the transducer, another part of the wave is simply reflected on the transducer surface and will start propagating away from the transducer as if it was another ultrasound transmission. This secondary “transmission” will propagate in a way similar to that of the original pulse, which means that it is reflected by the tissue and detected again (Figure 1.8, Video 1.3). These higher order reflections are called reverberations and give rise to ghost (mirror image) structures in the image (Video 1.4). These ghost images typically occur when strongly reflecting structures such as ribs or the pericardium are present in the image (Video 1.5). Similarly, as the reflected wave coming from the pericardium is very strong, its backscatter (i.e., propagating again towards the pericardium) will be sufficiently strong as well. This wave will reflect on the pericardium and can be detected by the transducer after the actual pericardial reflection arrives. In clinical practice, this causes a ghost image to be created behind the pericardial reflection that typically appears as a mirror image of the left ventricle around the pericardium in a parasternal long‐axis view. When complete reflections occur due to high acoustic impedance difference, no acoustic energy is transmitted to more distal structures and – as a consequence – no reflections from these distal structures can be obtained. As a result, a very bright structure will appear in the image followed by a signal void, i.e., an acoustic shadow (Video 1.6). For example, when a metallic prosthetic valve has been implanted, the metal (being very dense and extremely stiff) can cause almost complete ultrasound reflections, resulting in an apparently anechoic region distal to the valve. This occurs because no ultrasound energy reaches the deeper regions. Similarly, some regions in the image may receive little ultrasound energy due to superficial structures blocking ultrasound penetration. Commonly, ribs (being more dense and stiffer than soft tissue) are strong reflectors at cardiac diagnostic frequencies and can impair proper visualization of some regions of the image. Or, even more obviously, the absence of ultrasound gel may allow air to be in contact with the transducer. As air has a very different acoustic impedance from biological tissues, the acoustic wave (at the emission frequency in medical imaging) cannot propagate at all. These artifacts are most commonly referred to as “dropout” and can only be avoided by changing the transducer position/orientation. When signal dropout occurs at deeper regions only, the acoustic power transmitted can be increased. This will obviously result in more energy penetrating to deeper regions and will increase the overall signal‐to‐noise ratio of the image (in contrast to increasing the overall gain of the received signals as explained earlier). However, the maximal transmit power allowed is limited in order to avoid potential adverse biological effects. Indeed, at higher energy levels, ultrasound waves can cause tissue damage either due to cavitation (i.e., the formation of vapor cavities that subsequently implode and generate very high local pressures and temperatures) or tissue heating. The former risk is quantified in the mechanical index (MI) and should not pass a value of 1.9, while the latter is estimated through a thermal index (TI) and should not pass – depending on whether it is for nonobstetric or non‐neonatal applications – a value of a 1.0 for too long (the higher the value, the shorter the examination time should be). Overall, the acoustic power output of the system should not exceed 720 mW/cm2, in accordance with US Food and Drug Administration (FDA) recommendations. This value is verified by the regulatory bodies to allow manufacturers to enter the market and thus does not have to be verified by the operator. Both MI and TI are always displayed on the monitor during scanning and will increase with increasing power output. The operator has to find a compromise between image quality (including penetration depth) and the risk of adverse biological effects. In case penetration is not appropriate at maximal transmit power, the operator should choose a transducer with a lower transmit frequency. Figure 1.8 A transmitted wave (green) will reflect and result in an echo signal (green). The reflected wave will, however, partially reflect at the transducer surface (red) and generate secondary signals (red). Rather than mechanically moving or tilting the transducers, as in the early‐generation ultrasound machines, modern ultrasound devices use electronic beam steering. To do this, an array of piezoelectric crystals is used. By introducing time delays between the excitation of different crystals in the array, the ultrasound wave can be sent in a specific direction without mechanical motion of the transducer (Figure 1.9). The RF signal for a transmission in a particular direction is then simply the sum of the signals received by the individual elements. These individual contributions can be filtered, scaled, and time delayed separately before summing. This process is referred to as beam forming and is a crucial element for obtaining high‐quality images. The scaling of the individual contributions is typically referred to as apodization and is critical in suppressing side lobes and thus avoiding the associated artifacts. This concept can be generalized by creating a 2D matrix of elements that enables steering of the ultrasound beam in three dimensions. This type of transducer is referred to as a matrix array or 2D array transducer. Because each of the individual elements of such an array needs electrical wiring, manufacturing such a 2D array was technically challenging for many years because of the limitation of the thickness of the transducer cable. These obstacles have been overcome and 3D arrays are now available on high‐end ultrasound systems. Wave propagation as illustrated in Figure 1.2 only happens when the amplitude of the ultrasound wave is relatively small (i.e., the acoustic pressures involved are small). Indeed, when the amplitude of the transmitted wave becomes significant, the shape of the ultrasound wave will change during propagation, as illustrated in Figure 1.10. The phenomenon of wave distortion during propagation is referred to as nonlinear wave propagation. This wave distortion results in the generation of harmonic frequencies which are integer multiples of the transmitted frequency. Transmitting a 1.7‐MHz ultrasound pulse will thus result in the spontaneous generation of frequency components of 3.4, 5.1, 6.8, and 8.5 MHz, and so on. These harmonic components become stronger with propagation distance. The rate at which the waveform distorts for a specific wave amplitude is tissue dependent and characterized by the nonlinearity parameter, β (or the so‐called “B/A” parameter). The ultrasound scanner can be set up to receive only the second harmonic component through filtering of the received RF signal. If further post‐processing of the RF signal is done in exactly the same way as described earlier, a second harmonic image is obtained. Such an image typically has a better signal‐to‐noise ratio by avoiding clutter noise due to (rib or air) reverberation artifacts. As second harmonic waves are increasingly present with increasing depth, second harmonic imaging results in better images at deeper depths. This harmonic image is commonly used in patients with poor acoustic windows and poor penetration. Although harmonic imaging increases the signal‐to‐noise ratio, it has intrinsically poorer axial resolution as further discussed later in this chapter. Higher harmonics (i.e., third, fourth, etc.) are also present but typically fall outside the bandwidth of the transducer, which is the range of analyzable frequencies, and thus are not detected by the transducer. Harmonic imaging has become the default cardiac imaging mode for adult scanning on many systems. It is typically unnecessary to use harmonic imaging in young infants as it reduces axial resolution. Switching between conventional and harmonic imaging is done by changing the transmit frequency of the system. For lower frequency transmits, the default setting is usually a harmonic imaging mode, which is indicated on the display by showing both transmit and receive frequencies (e.g., 1.7/3.4 MHz). When a single frequency is displayed, the scanner is in a conventional (i.e., fundamental) imaging mode. For pediatric scanning, especially in smaller infants, fundamental imaging is the preferred mode due to its better spatial resolution, and presets for higher frequency probes are typically programmed in the fundamental frequency. Figure 1.9 An array of crystals can be used to steer the ultrasound beam electronically by introducing time delays between the activation of individual elements in the array. Figure 1.10 Nonlinear wave behavior results in changes in shape of the waveform during propagation. As blood is a poor reflector of ultrasound energy (i.e., relative homogeneity of the acoustic impedance), it shows dark in the image. For some applications, like myocardial perfusion assessment, it can be useful to artificially increase blood reflectivity. This can be achieved by using an ultrasound contrast agent. As air is a very strong reflector of ultrasound energy due its relative high compressibility and low density when compared with soft tissue, it often is used as a contrast agent. The injection of small air bubbles with diameters similar to those of red blood cells, significantly increases blood reflectivity. Agitated saline can be used or ultrasound contrast agents that contain encapsulated air bubbles to limit the diffusion of air in blood. Contrast imaging can be helpful for visualizing the endocardial border as it enhances the difference in gray value between the myocardium and the blood pool. It can be used in patients with poor image penetration to better visualize the endocardial border. Contrast injection can also be used for detecting shunts. As in pediatrics, right‐to‐left shunting can be present; typically carbon dioxide is the preferred gas to create agitated saline. Contrast agents can also be used to increase the brightness of the perfused myocardial tissue, although artifacts can be present which can make the interpretation of the perfusion images less obvious. At present, different contrast agents are commercially available for clinical use but regulatory approval for pediatric use differs between countries. In the presence of right‐to‐left shunting, contrast agents should be used cautiously and only by experienced operators. Resolution is defined as the shortest distance at which two adjacent objects can be distinguished as separate. The spatial resolution of an ultrasound image varies depending on the position of the object relative to the transducer. Also, the resolution in the direction of the image line (range or axial resolution) is different from the one perpendicular to the image – within the 2D image plane (azimuth or lateral resolution) – which is different again from the resolution in the direction perpendicular to the image plane (elevation resolution). In order to obtain an optimal axial resolution, a short ultrasound pulse (high frequency) needs to be transmitted. The length of the transmitted pulse is mainly determined by the characteristics of the transducer, i.e., the range of frequencies that can be generated/detected – referred to as its bandwidth. The bandwidth is most commonly expressed relative to the center frequency of the transducer. A typical value would be 80%, implying that for a 5 MHz transducer the absolute bandwidth is about 4 MHz. This type of transducer can thus generate/receive frequencies in the range of 3–7 MHz. The absolute transducer bandwidth is typically proportional to the mean transmission frequency. A higher frequency transducer will thus produce shorter ultrasound pulses and, thus, better axial resolution. Unfortunately, as discussed previously, higher frequencies are attenuated more by soft tissue and are impacted by depth. As such, a compromise needs to be made between image resolution and penetration depth. In pediatric and neonatal cardiology where the acquisition depth is less, higher frequency transducers can be used to increase image spatial resolution. Typically for infants 10–12 MHz transducers are used, resulting in a typical axial resolution of the order of 150 μm, knowing that the axial resolution is roughly equal to λ. Most systems allow changing the transmit frequency of the ultrasound pulse within the bandwidth of the transducer. As such, a 5‐MHz transducer can be used to transmit a 3.5‐MHz pulse which can be practical when penetration is not sufficient at 5 MHz. The lower frequency will result in a longer transmit pulse with a negative impact on axial resolution. Similarly, for second harmonic imaging, a narrower band pulse needs to be transmitted, as part of the bandwidth of the transducer needs to be used to be able to receive the second harmonic. As such, in harmonic imaging mode, a longer ultrasound pulse is transmitted (i.e., less broad band) resulting in a worse axial resolution of the second harmonic image despite improvement of the signal‐to‐noise ratio. Therefore, some of the cardiac structures appear thicker, especially valve leaflets. This should be considered when interpreting the images. Lateral resolution is determined by the width of the ultrasound beam (i.e., the width of the main lobe). The narrower the ultrasound beam, the better the lateral resolution. In order to narrow the ultrasound beam, several methods can be used but the most obvious one is focusing. This is achieved by introducing time delays between the firing of individual array elements (similar to that done for beam steering) in order to make sure that the transmitted wavelets of all individual array elements arrive at the same position at the same time and will thus constructively interfere (Figure 1.11). Similarly, time delaying the reflections of the individual crystals in the array will make sure that reflections coming from a particular point in front of the transducer will sum in phase and therefore create a strong echo signal (Figure 1.11). Because the sound velocity in soft tissue is known and is considered homogeneous, the position from which reflections can be expected is known at each time instance after transmission of the ultrasound pulse. As such, the time delays applied in receive can be changed dynamically in order to move the focus point to the appropriate position. This process is referred to as dynamic (receive) focusing. In practice, dynamic receive focusing is always used and does not need adjustments by the operator, in contrast to the transmit focus point whose position should be set manually. Obviously, to resolve most morphologic detail, the transmit focus should always be positioned close to the structure/region of interest. Most ultrasound systems allow the selection of multiple transmit focal points. In this setting, each image line will be created multiple times with a transmit pulse at each of the set focus positions, and the resulting echo signals will be combined in order to generate a single line in the image. Although this results in a more homogeneous distribution of the lateral resolution with depth, it takes more time to generate a single image and thus will result in lowering the frame rate (i.e., temporal resolution). Figure 1.11 Introducing time delays during the transmission of individual array elements (left) allows for all wavelets to arrive at a particular point (focus) simultaneously. Similarly, received echo signals can be time delayed so that they constructively interfere (receive focus). The easiest way to improve the focus performance of a transducer is by increasing its size (i.e., aperture). Unfortunately, the footprint needs to fit between the patient’s ribs, thereby limiting the size of the transducer and thus limiting lateral resolution of the imaging system. This parameter is essential for phased‐array probes used in cardiology, which have a small aperture in order to thwart anatomic obstacles (ribs). Ultimately, the lateral resolution depends on the wavelength and shape of the wave dictated by the geometry of the probe and/or by the emission parameters (i.e., time delays) of the wave beam. As an example, for an 8‐MHz pediatric transducer, realistic numbers for the lateral resolution of the system are depth dependent and are approximately 0.3 mm at 2 cm, going up to 1.2 mm at 7 cm depth. For elevation resolution, the same principles hold as for lateral resolution in the sense that the dimension of the ultrasound beam in the elevation direction will be determinant. However, most ultrasound devices are still equipped with 1D array transducers. As such, focusing in the elevation direction needs to be done by the use of acoustic lenses (similar to optic lenses, acoustic lenses concentrate energy in a given spatial position), which implies that the focus point is fixed in both transmit and receive (i.e., dynamic focusing is not possible in the elevation direction). This results in a resolution in the elevation direction that is worse than the lateral resolution. The homogeneity of the resolution is also worse with depth. Moreover, the transducer aperture in the elevation direction is typically somewhat smaller (in order to fit in between the ribs of the patient) resulting in a further decrease of elevation resolution compared with the lateral component. Newer systems with 2D array transducer technology have more similar lateral and elevation image resolution. Matrix‐array transducers not only create 3D images but also allow the generation of 2D images of higher/more homogeneous spatial resolution. By definition, temporal resolution in medical imaging is the number of images obtained per second (i.e., frame rate). This parameter should be differentiated from the transmission/reception frequency of the acoustic wave (i.e., pulse repetition frequency, PRF). In conventional ultrasound imaging, which requires focused transmissions (as described later), a large number of transmissions/receptions must be repeated before an image can be obtained, so the frame rate is much lower than the PRF. Typically, a 2D pediatric cardiac image consists of 300 lines. The construction of a single image thus takes about 300 × 100 μs (the time required to acquire one line) or 30 ms. In this example, the PRF is 10,000 Hz while the frame rate is 33 Hz. Thus, 33 images can be produced per second, which is sufficient to look at motion (e.g., old television displays based on cathode ray tubes only displayed 25 frames per second). With more advanced imaging techniques such as parallel beam forming, higher frame rates can be obtained (70–80 Hz). In order to increase frame rate further, either the field of view can be reduced (i.e., a smaller sector will require less image lines to be formed and will thus speed up the acquisition of a single frame) or the number of lines per frame (i.e., the line density) can be reduced. The latter comes at the cost of spatial resolution, as image lines will be further apart. There is thus an intrinsic trade‐off between the image field of view, spatial resolution, image contrast, and temporal resolution. Most systems have a “frame rate” button nowadays that allows changing the frame rate, although this always comes at the expense of image quality. Higher frame rates are important when the heart rate is higher as is often the case in pediatric patients and when studying short‐lived events (e.g., isovolumetric contraction) or fast‐moving structures (e.g., valve leaflets). In order to increase the frame rate for a given PRF, several techniques can be applied, including those described in the previous section. Figure 1.12 Conventional versus high frame rate ultrasound. (a) Traditional echocardiography makes use of focused transmit beams. (b–d) High frame rate imaging either transmits several focused beams in parallel (two in the example in (b)); unfocused or plane waves (c); or defocused/diverging waves (d). For the high frame rate imaging techniques, image lines are created in the insonified region by receive beam forming. Figure 1.13 Potential applications of ultrafast ultrasound imaging in pediatric and congenital cardiology. Overall, for both plane/diverging wave and multiline transmit imaging, a compromise needs to be found between image quality and frame rate, as in conventional imaging. However, for these high frame rate modes, this compromise gets intrinsically skewed towards higher frame rates. In general, for comparable frame rates, both high frame rate methodologies have been shown to be very competitive and choosing one or the other approach may mostly be determined by how easily it can be implemented on a given system (given its electronic hardware constraints). To date, these high frame rate solutions are not commercially available although this is likely to change in the near future. For a more elaborate discussion of these novel imaging modalities and their (potential) clinical use, the reader is referred to a review by Cikes et al. [4]. All the principles mentioned so far can be used to optimize image acquisition for the pediatric population. As children are smaller, less penetration is required. The heart rates are higher, requiring higher temporal resolution and, as structural heart disease is more common in the pediatric age group, spatial resolution needs to be optimized to obtain the best possible diagnostic images. Image optimization will always be a compromise between image quality and temporal resolution. A few general recommendations can be made which can help in image optimization: When an acoustic source moves relative to an observer, the frequencies of the transmitted and the observed waves are different. This phenomenon is known as the Doppler effect. A well‐known example is that of an ambulance passing a static observer: the observed pitch of the siren is higher when the car approaches than when it moves away. The Doppler phenomenon can be used to measure tissue and blood velocities by comparing the transmitted with the received ultrasound frequency. Indeed, when ultrasound scattering occurs at stationary tissues, the transmitted and reflected frequencies are identical. This statement is only true when attenuation effects are negligible. In soft tissue there will be an intrinsic frequency shift due to frequency‐dependent attenuation. When scattering occurs at tissues in motion (Figure 1.14), a (additional) frequency shift – the Doppler shift (fD) – will be induced that is directly proportional to the velocity (v) by which the tissue is moving: Figure 1.14 The Doppler effect will induce a frequency shift of the transmitted ultrasound wave when the reflecting object is in motion. T, transmitter.
CHAPTER 1
Ultrasound Physics
Physics and technology of echocardiography
How the ultrasound image is created
The pulse–echo experiment
Grayscale encoding
Image construction
Image artifacts
Side lobe artifacts
Reverberation artifacts
Shadowing and dropout artifacts
Ultrasound technology and image characteristics
Ultrasound technology
Phased‐array transducers
Second harmonic imaging
Contrast imaging
Image resolution
Axial resolution
Lateral resolution
Elevation resolution
Temporal resolution
High frame rate imaging
Image optimization in pediatric echocardiography
Doppler imaging
Continuous‐wave Doppler
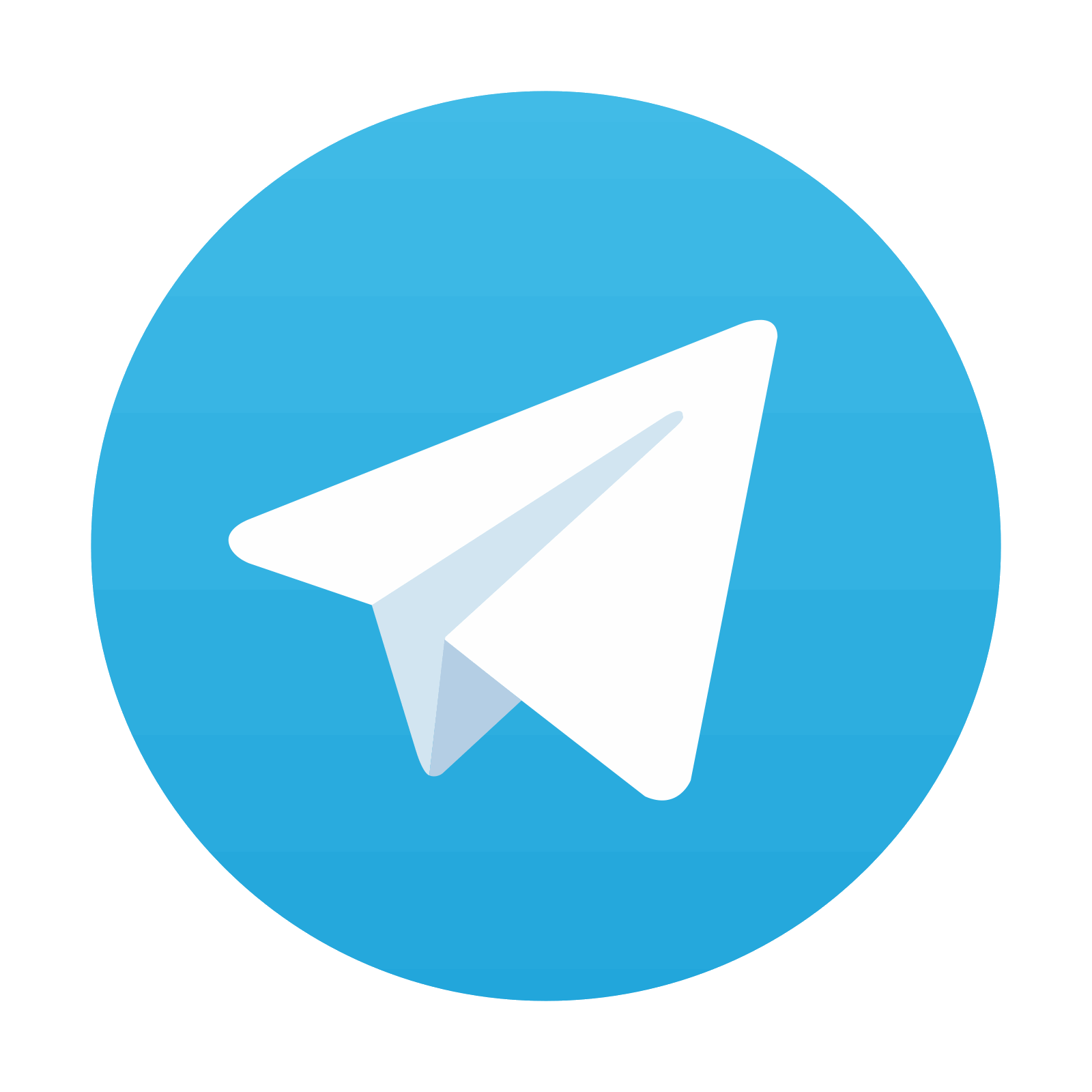
Stay updated, free articles. Join our Telegram channel
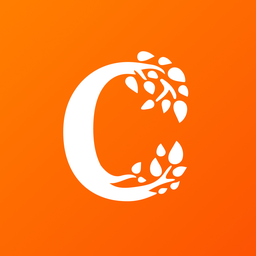
Full access? Get Clinical Tree
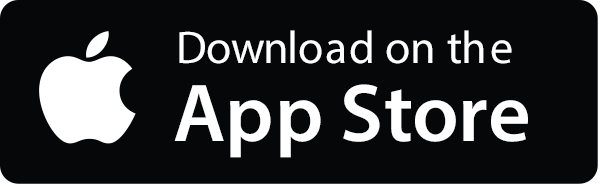
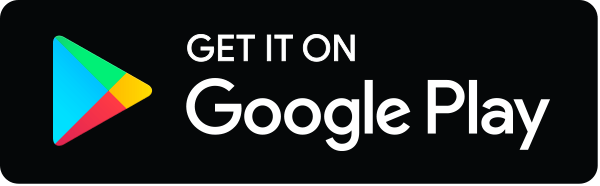