Chapter 14 Visual System
EMBRYOLOGY OF THE EYEBALL
The eyeball (bulbus oculi) is derived from neurectoderm (retina), surface ectoderm (lens and cornea), and mesoderm-neural crest (cornea, sclera, and uvea). In the bird, ectodermal neural crest contributes to the cornea, sclera, and uvea.152 The amount of contribution of neural crest in the mammal remains to be determined.
The neurectodermal contribution to the eyeball is induced in the neural plate stage at its rostral end on the median plane just before closure to form the neural tube. This portion of the neural tube is destined to form the prosencephalon and subsequently the diencephalon. This initially single primordial optic field area (eye field) is influenced by the adjacent head mesenchyme, which includes mesoderm and neural crest cells. This portion of head mesenchyme is rostral to the notochord and is therefore designated prechordal mesenchyme. The morphogenesis of the eye is controlled by the Pax 6 gene that is expressed by the prechordal mesenchymal and neurectodermal eye fields. Sonic hedgehog is the signaling protein secreted by these cells that has a diverse role in this morphogenesis.222 This signaling molecule plays a critical role in establishing the bilateral eye fields from the initial single optic field area of neurectoderm. This area first appears as two depressions within the neural tube, the optic pits, in each half of the initial prosencephalon. They proceed to grow laterally as evaginations from the neural tube known as optic vesicles (Fig. 14-1). Evidence in lower vertebrates indicates that the prosencephalic cells that contribute to each optic vesicle come from both sides of the prosencephalon.99 This arrangement may relate to the subsequent development of optic nerve axon crossing at the optic chiasm. Improper separation of the single optic area into two primordia by the prechordal mesenchyme leads to the development of the cyclopic malformation with a single median-plane eyeball. The Veratrum sp. cyclopamine alkaloid ingested by the ewe interferes with the action of the sonic hedgehog molecule which results in the cyclopic malformation. This occurs specifically at 14 days of gestation.57 See Chapter 3 for a description of this malformation with the brain malformation holoprosencephaly.
The optic vesicles bulge laterally from the prosencephalon and lie adjacent to the surface ectoderm, where their cells proliferate and induce the surface ectoderm to proliferate to form the lens placode. Differential growth in the wall of the optic vesicle results in the invagination of the portion of the optic vesicle adjacent to the lens placode. This infolding and the more rapid growth of the outer wall of the optic vesicle result in the formation of an optic cup. As the optic vesicle cup grows away from the prosencephalon, its connection elongates into the optic stalk, which is the precursor of the pathway of the optic nerve. The optic cup is incomplete ventrally where the optic or choroidal fissure forms. The embryonic hyaloid vasculature enters the developing eye through this fissure. In time, fusion of the edges of this fissure will close the fissure, except for a small notch. The infolding that produces the optic cup also involves the optic stalk, which provides a pathway for the optic nerve axons to enter the brain. The two walls of the optic cup differentiate into the retina.
As the optic vesicle invaginates to form the optic cup, the lens placode that is developing in the surface ectoderm also invaginates to form the lens vesicle, which ultimately separates from the overlying surface ectoderm and remains located at the opening of the optic cup. A fine fibrous connection remains between the inner wall of the optic cup and the posterior surface of the lens vesicle. The anterior cells of the lens vesicle remain cuboidal. The posterior cells elongate to form the primary lens fibers that eventually obliterate the cavity of the lens vesicle. These primary lens fibers lose their nuclei and become the embryonic lens nucleus. The anterior cuboidal cells remain as the lens epithelium. Proliferation and elongation of these cells around the equator of the lens gives rise to secondary lens fibers. These fibers grow anteriorly between the anterior epithelium and the embryonic nucleus and posteriorly over the embryonic nucleus beneath the lens capsule. Secondary fibers are continually formed at the equator for the life of the animal. The characteristics of these lens fibers impart transparency to the lens. Congenital cataracts develop in the primary lens fibers of the embryonic lens nucleus.7
As these primordial ectodermal structures are forming internal components of the eyeball, a component of mesodermal-neural crest is added to the external surface of the optic stalk and cup. This structure can be considered as an extension of the two supporting layers that are formed around the entire central nervous system, the dense protective dura, and the thinner vascular pia-arachnoid (Fig. 14-2).
As the loose mesoderm-neural crest surrounding the brain differentiates into the vascular pia-arachnoid (leptomeninges), this process of differentiation continues along the optic stalk and over the optic cup and lens vesicle. The subarachnoid space found between the pia and the arachnoid over the brain continues along the optic stalk to the posterior surface of the optic cup where it stops. This subarachnoid space is implicated in the spread of disease between the brain and the eyeball. At the optic cup, this space is obliterated, and the mesoderm-neural crest, homologous with the pia-arachnoid, differentiates into the uveal coat of the eyeball (choroid, ciliary body, and iris) and forms the endothelial layer on the posterior (inner) surface of the cornea. This initially loose mesoderm-neural crest completely fills the area between the lens vesicle and the corneal endothelium. A space forms in this tissue, leaving the mesoderm-neural crest anteriorly as the corneal endothelium and posteriorly as the body of the iris and pupillary membrane. This space is the anterior chamber, which fills with aqueous humour. The pupillary membrane is the mesoderm-neural crest located centrally over the lens. Ultimately, it disintegrates to form the pupil. Abnormalities in this disintegration result in a persistent pupillary membrane. This condition is thought to be inherited in Basenji dogs.139,172 The space formed in the mesoderm-neural crest between the iris and the lens is the posterior chamber, which also fills with aqueous humour. The uvea is the vascular tunic of the eyeball that at the optic stalk is continuous with the pia-arachnoid, the vascular tunic of the central nervous system (CNS). The cells of these layers have some similar histologic characteristics.
The space between the inner wall of the posterior portion of the optic cup and the posterior surface of the lens is the vitreous chamber. The vitreous body that fills this space is derived from secretions from the optic cup, the lens, and the enclosed mesenchyme. Hyaloid blood vessels course in the mesoderm from the base of the brain along the optic stalk in the optic fissure to supply the optic cup, vitreous, and lens. Branches of these blood vessels course through the vitreous to the posterior surface of the lens. These hyaloid vessels normally disappear after birth. Remnants may persist in dogs to around 4 months of age and in cattle until 1 year or more. These reminants are seen emerging from the optic disk that forms at the site of the optic stalk. Abnormal persistence of this hyaloid vasculature to the lens results in the development of cataracts of the posterior lens capsule and an accumulation of blood pigment and fibrovascular tissue posterior to the lens. This lesion is called persistent hyperplastic tunica vasculosa lentis and persistent hyperplastic primary vitreous.120,159,168 A hereditary basis has been suggested for this disorder in the Doberman pinscher.194
Differentiation of the optic cup is similar to the differentiation of the neural tube, with two adjacent walls separated by a neural canal (Fig. 14-3; see also Fig. 14-2). The wall of the optic vesicle originally consisted of a single layer of columnar neuroepithelial cells, similar to any other component of the neural tube. These cells were rapidly proliferating neuroepithelial cells. As the lumen of the vesicle is reduced to a small space by the infolding of the anterior surface, this area forms an outer and inner wall to the optic cup. The differentiation of each of these walls can be compared with that of the neural tube, with the formation of germinal (ventricular zone), mantle, and marginal layers. The entire outer wall of the optic cup, which initially proliferated, later regresses to a single layer of cells. Posterior to where the ciliary body will form and adjacent to the choroid, this optic cup outer wall is the pigment layer of the retina. Anterior to this, it forms the outer layer of the two cell layers that cover the posterior surface of the ciliary body (pars ciliaris retina) and iris (pars iridica retina). Thus this differentiated outer wall of the optic cup is homologous to the ependymal layer of the differentiated neural tube that lines the ventricular system and the central canal and forms the epithelial portion of the choroid plexuses (see Chapter 3). The inner wall of the optic cup, posterior to the level of the ciliary body, differentiates into the multilayered sensory portion of the retina, the pars optica retina. Anterior to this structure, the inner wall of the optic cup differentiates into a single layer of cells that joins with the single layer of cells of the outer wall of the optic cup to form the two-cell layer that covers the posterior surface of the ciliary body (pars ciliaris retina) and the iris (pars iridica retina). Thus these two layers of cells derived from the outer and inner walls of the optic cup are called the pars ciliaris retina and pars iridica retina, named for the structures that they cover on the posterior surface. The rostral extent of the pars iridica retina determines the margin of the iris, which is the border of the pupil. Beyond this structure, the mesoderm-neural crest of the pupillary membrane degenerates to produce the pupillary space, the pupil. Improper degeneration of this membrane leaves persistent remnants, which may be an inherited abnormality in the Basenji breed.
In the inner wall of the optic cup posterior to the ciliary body, the neuroepithelial cells proliferate as the germinal layer (see Fig. 14-3). With development, this germinal layer differentiates into three layers of neurons. The outer layer of cells closest to the choroid and sclera forms the photoreceptor neurons. These neurons are separated from the pigment epithelium of the retina by the collapsed slitlike lumen of the neural canal and are homologous to the ependymal layer of the nervous system. Two other layers of neurons differentiate in this inner wall of the optic cup. These layers include the layer of bipolar neurons and the ganglion neuron layer. This latter name is another inappropriate use of the term ganglion, given that this collection of neuronal cell bodies is within the CNS. Nevertheless, the name has been retained as the acceptable term. The combined layer of bipolar neurons and the ganglion neuronal layer are homologous to the mantle layer of the neural tube. The nerve fiber layer is a layer of axons from the ganglion cell neurons located on the inner surface of this inner wall facing the vitreous. This layer is homologous to the marginal layer on the surface of the neural tube. The axons of these ganglion neurons course to the optic stalk and grow through it to the brain to form the optic nerve. As these axons penetrate the optic stalk, some optic stalk cells degenerate; others differentiate into the glial cells of the optic nerve. The oligodendroglia of the optic stalk myelinate the axons of the ganglion neurons. Myelination occurs in the optic nerve from the brain to the eyeball. This feature is apparent in the white color of the optic disk seen with the ophthalmoscope in the back of the eyeball. The area seen with this instrument is the fundus of the eyeball. No myelination occurs in the nerve fiber layer of the retina.
In dogs and cats at birth, the single layer of ganglion neurons is separated from an outer thick layer of undifferentiated cells that represent the primordia of the photoreceptor neurons and the bipolar neurons.65,100,187,196 These neuronal cell bodies become separated by the external plexiform layer in the first 7 days of life so that the three definitive layers of retinal neurons are established. In retinal development, an overproduction of neurons occurs, which leads to significant apoptosis to attain the normal population by a few weeks after birth. Their normal development and survival is dependent on these neurons forming synapses on neurons in the brain (i.e., lateral geniculate nucleus, rostral colliculus, pretectal nucleus).222 Ganglion neurons that do not make appropriate synapses degenerate by apoptosis. In the chicken, up to 20% of the ganglion neuron population degenerates in normal retinal development.95 In the fetal kitten there are approximately 600,000 ganglion neurons at 47 days of gestation. These neurons progressively decrease to the permanent number of approximately 150,000 by 2 weeks after birth. The histologically mature retina is apparent by 6 weeks of age in the dog, which coincides approximately with the development of visual function. The various media of the eyeball are not transparent until 5 to 6 weeks after birth. The following sequence of development of the eye has been observed in the dog.6,65
In contrast to the dog with its short gestational period, the bovine eyeball is fully developed at birth. Studies have shown that the bovine eyeball appears well developed by the end of the second trimester of gestation. The following sequence of development has been observed in the bovine embryo-fetus.20
HISTOLOGY OF THE PARS OPTICA RETINA
Ten layers of the pars optica retina are described here, progressing from the outer (scleral) surface to the inner (vitreal) surface. These layers include three groups of neurons, two types of interneurons, astrocytes, and numerous neurotransmitters (Fig. 14-4).156,186,187
Pigment Epithelium of the Retina
This epithelium is composed of a single layer of cuboidal cells with their base on a basement membrane apposed to the choroid and their apex facing the photosensitive layer across the potential space of the neural tube (lumen of the optic cup).159,217 This epithelium is entirely derived from the outer wall of the optic cup. Numerous processes of the apical cell membrane and cytoplasm interdigitate with the processes of the external segments of the photoreceptor rods and cones. In cats, this structure is quite elaborate, with specialized sheetlike projections from the apical surface of the pigment epithelium ensheathing the external segments of the cones and, to a lesser degree, the rods. This close arrangement may facilitate the role of the pigment epithelium in the regeneration of rhodopsin, the visual pigment in the rods, and the removal of degenerate rod membranous lamellae by pigment-cell phagocytosis. Many melanin granules fill the apical cytoplasm of the pigment cells throughout the retina, except over the area occupied by the tapetum lucidum in the adjacent choroid. The presence of these pigment granules partly accounts for the dark color of the non-tapetal portion of the fundus observed with the ophthalmoscope. The tapetum lucidum in the choroid is rendered more visible by the absence of melanin pigment in this pigment epithelium. These pigment cells and the photoreceptor neurons are nourished by the vessels of the choriocapillaris layer of the choroid. This layer is adjacent to the scleral side of the pigment epithelial cells. Exchange of substances between these choroidal vessels and the photoreceptor neurons must pass through the pigment epithelial cells.
Photosensitive Layer
The photosensitive layer,2 divided into two segments, represents the dendritic zone and cell body of the special somatic afferent neuron, the photoreceptor cell.210,217 The external and internal segments of the layer are composed of modifications of the cell processes and the bodies of the rod and cone cells. Their nuclei are in the external nuclear layer. The external segment of the photosensitive layer represents the dendritic zones of these neurons. For each neuron (rod and cone), the component in the external segment consists of parallel lamellae within an elongate cell process. These lamellae are orderly stacks of flattened, double-membrane sacs in the form of disks. These membranes are oriented transversely to the axis of the cell process. They are formed at the base of the external segment from the modified cilium located there. These membranes are continually being produced. They migrate distally (away from the vitreous) and are cast off at the outer portion, where they are phagocytized by the pigment epithelial cells.73,131,220 In the rods, these membranes contain the visual pigment rhodopsin, the photoreceptor substance responsible for light absorption and the initiation of the visual stimulus. A similar substance, iodopsin, is in the cone membranous lamellae. The rod cells are sensitive to low levels of illumination (night vision) and are more abundant in nocturnal animals. Cone cells respond to high levels of illumination (day vision) and are responsible for initiating color vision.
External Plexiform Layer
The external plexiform layer122 is composed of the axons and telodendria of the photoreceptor neurons and the axons and dendritic zones of the bipolar neurons and their synaptic arrangements. Intermingled with these neuronal processes are the processes of horizontal cells. The horizontal cell is an interneuron transmitting between different groups of photoreceptor neurons.
Internal Plexiform Layer
The internal plexiform layer123,124 is composed primarily of the axons and telodendria of the bipolar neurons and the axons and dendritic zones of the ganglion neurons and their synaptic arrangements. In addition, the processes of the amacrine interneurons extend throughout this layer.
Ganglion Layer
This neuronal ganglion layer contains the cell bodies and nuclei of the third neuron in the visual pathway.* These cell bodies and nuclei are large multipolar neurons with a large cell body and nucleus that comprise an incomplete layer one to two cells thick between the internal plexiform layer and the nerve fiber layer. This neuron transmits the visually induced impulse to the brain by way of its axon in the optic nerve. Endoplasmic reticulum, Nissl substance, is evident in the cytoplasm of these neuronal cell bodies. These cell bodies vary in size from 6 to 35 microns in diameter. The smallest cell bodies have axons that project to the rostral colliculus. The larger cell bodies have axons that project mostly to the lateral geniculate nucleus. Functional differences may be found between the medial (nasal) and lateral (temporal) portions of the retina. Mean ganglion neuron size is greater in the lateral retina than in the medial retina, suggesting a greater projection from the lateral retina (medial visual field in space) to the lateral geniculate nucleus of the thalamus for cerebral projection. An increased number of ganglion neurons can be found in the area centralis, where the smaller cell bodies predominate. Remember that the term for this layer, ganglion, is a misnomer because it implies that these neurons are outside the CNS, which is incorrect.
Internal Limiting Membrane
Figs. 14-5 and 14-6 show examples of these layers in a dog and horse eye.
Area Centralis
Humans have a round area for most distinct vision located dorsolateral to the optic disk known as the macula (spot), fovea, or central area. This portion of the retina has the highest resolving power necessary for acute vision. In this area, the retina is composed of only cone cells in the photoreceptor layer, and other modifications occur to facilitate its function for visual acuity. For most precise close-up vision such as reading extraocular neuromuscular mechanisms are responsible for the visual field being focused on this central area in each eyeball. Domestic animals have various modifications of this area. In no species of domestic animal is this area readily seen with the ophthalmoscope, except possibly in some cats. In the cat, this area may be identified as a pale streak or oval in the area of the tapetum lucidum dorsolateral to the optic disk in which the blood vessels (arterioles) converge (Fig. 14-7).209 The area itself is devoid of any large blood vessels. In this area centralis in the cat, an increase in the number of cone cells relative to rod cells can be found in the photosensitive layer, with an overall increase in thickness of the external nuclear layer. The length of the external segments of the photoreceptor cells is increased. The bipolar and ganglion neurons in this area are increased in number as well. The axons in the nerve fiber layer form an arc as they leave the area centralis and course to the optic disk. If the area centralis is defined as a region of the pars optica retina in which ganglion neuron density increases to a peak, then, in all ungulates, this is evident as a streak of high cell density extending horizontally dorsal to the optic disk. This increase in ganglion neurons is maximal near the lateral end of the visual streak, which corresponds approximately to the location of area centralis in cats.
Optic Disk
The optic disk at the origin of the optic nerve varies in shape in the domestic animals, as well as between breeds of dogs, from round to oval to triangular.15,141,218 It is usually slightly ventrolateral to the posterior pole of the eyeball and varies in its relationship to the level of the tapetum lucidum. In toy breeds, the optic disk may be in the retina entirely inferior to the inferior border of the tapetum lucidum. In medium-size breeds, it is usually halfway over the inferior border of the tapetum lucidum. In large breeds, it may be entirely over the area of the tapetum lucidum. In cats, the optic disk is small, round, and always over the area of the tapetum lucidum. In horses and ruminants, it is just inferior to the inferior border of the tapetum lucidum. The degree of myelination of the optic disk varies between species of domestic animal, with it being the most developed in the dog and least in cattle. The size and arrangement of the blood vessels at the optic disk also vary between species and are the least prominent in the horse and limited to the periphery of the optic disk. Some examples of photographs of the fundus of a normal dog, cat, cow, and horse can be found in Figs. 14-8 through 14-11.
CENTRAL VISUAL PATHWAY
Optic Nerve
The growth of the ganglion neuronal axons through the embryonic optic stalk produces the optic nerve.* In reality, the term optic nerve is a misnomer, given that a nerve is defined as a bundle of neuronal axons outside the CNS and myelinated by Schwann cells. The optic nerve is, in fact, a tract of the CNS based on its origin within the optic cup, which is an extension of the rostral portion of the neural tube that forms the prosencephalon. The axons in the optic nerve are myelinated by oligodendroglia, and this nerve contains astrocytes. The optic nerve is surrounded by meninges and a subarachnoid space. Extensions of the pia course through the nerve as septa. This structure has clinical importance because the optic nerve is subject to diseases of the CNS and not the peripheral nervous system. When contrast is injected into the cerebellomedullary cistern to produce a myelogram and the head is lowered to direct the contrast into the cranial cavity, an encephalogram is produced. The contrast in the subarachnoid space around the brain surrounds the optic nerve from the middle cranial fossa rostrally to the posterior surface of the eyeball, forming an optic thecogram.
The optic nerves course caudally in the orbit surrounded by their meninges, extraocular muscles, and periorbita. They enter the skull through the optic canals of the presphenoid bone, which have considerable length in the horse. The optic nerves join at the optic chiasm ventral to the rostral aspect of the hypothalamus and rostral to the hypophysis.
Optic Chiasm and Tract
At the optic chiasm in domestic animals, a majority of the axons in each optic nerve cross to enter the opposite optic tract. These axons are destined to influence the contralateral occipital lobes of the cerebral hemispheres (Fig. 14-12).31,37,89,188 This arrangement corresponds to the pattern that most sensory modalities that can be localized in space (general proprioception, general somatic afferent) are represented contralaterally in the brain. The factor that determines whether ganglion neuronal axons cross or not in the chiasm is the subject of extensive ongoing investigations. Research has shown that the signal molecule, sonic hedgehog, that is produced in retinal ganglion neurons plays a role in this development.
In fish and birds, all of the optic nerve axons cross in the optic chiasm. In mammals, partial decussation develops in relationship to the development of a binocular field of vision, with frontal positioning of the eyes and the ability to perform coordinated conjugate movements of the eyeballs, including convergence. In primates, in whom this feature is most developed, the degree of decussation is slightly over 50%. Estimates suggest that the degree of decussation in the cat is 65%; in dogs, 75%; and in the horse and farm animals, 80% to 90%. Based on this information, the cat most closely resembles the primate in degree of decussation, frontal positioning of the eyes, and presumably conjugate movements of the eyeballs. As the visual system becomes more complex along with the capability for binocular vision, decussation in the optic chiasm decreases. In avian species, decussation is 100%. The Siamese cat and white Persian tiger have been found to have an increased number of axons that cross in the optic chasm, which is reflected in an altered lamination of the lateral geniculate nucleus. Congenital pendular nystagmus and a convergent strabismus have been considered to be related to this altered visual system architecture in these species.* This feature was described in Chapter 12.
The axons that cross in the optic chiasm come from ganglion neurons in the medial (nasal) aspect of the retina (see Fig. 14-12).197,200 Axons from ganglion neurons in the lateral (temporal) aspect of the retina remain ipsilateral in their course through the central visual pathway. Neuroanatomic studies in the cat have determined that this division between medial and lateral portions of the retina, based on the axons that cross in the chiasm, is a vertical line approximately through the area centralis. This arrangement was initially determined by cutting one optic tract and studying the retrograde degeneration that occurred in the ganglion neurons of the retina, the axons of which were severed (Fig. 14-13). Studies using horseradish peroxidase injected into the lateral geniculate nucleus of the thalamus have further confirmed this nasotemporal division of the retina.
The optic tracts course caudodorsolateral over the side of the diencephalon, progressing from ventral to lateral to caudal in relationship to the internal capsule to reach the lateral geniculate nucleus.19 This nucleus forms a caudodorsolateral protrusion of the thalamus (see Fig. 2-2 through 2-6). Each optic tract contains axons mostly from the medial retina of the contralateral eyeball and the lateral retina of the ipsilateral eyeball. The visual field is defined as the area in space observed by each eyeball when fixed at any one moment. Therefore light from the lateral half of the visual field of each eyeball will stimulate the medial retina, and light from the medial half of the visual field will stimulate the lateral retina. Thus each optic tract contains the axons of neurons in which light generates an impulse from the same half of the visual field of each eyeball. That is, the left optic tract contains the axons of neurons stimulated by light from the right half of the visual field of the left and right eyeballs. Objects in the right visual field of each eyeball are therefore represented in the left central visual pathways.
Pathway for Conscious Perception— Visual Cortex
Approximately 80% of the optic tract axons in the cat terminate in the lateral geniculate nucleus (see Figs. 2-4 through 2-6). This nucleus contains neuronal cell bodies organized in specific laminae.* A retinotopic anatomic relationship is maintained throughout the central visual pathway. This relationship is reflected in the laminar pattern of the lateral geniculate nucleus. The axons of the neuronal cell bodies in the lateral geniculate nucleus project into the internal capsule and course caudally as the optic radiation in the caudal limb of the internal capsule, which forms the lateral wall of the lateral ventricle (see Figs. 2-5, 2-6). These axons terminate in the cerebral (visual) cortex on the lateral, caudal, and medial aspects of the occipital lobe.72,150 The gyri that comprise this area include the caudal part of the marginal, ectomarginal (laterally), occipital (caudally), and the splenial (medially) gyri. This pathway from the optic tract to the lateral geniculate nucleus to the optic radiation of the internal capsule to the visual cortex must be intact for normal conscious visual perception to occur.
Reflex Pathway
For the pupillary light reflex, approximately 20% of the optic tract axons in the cat pass over the lateral geniculate nucleus to terminate in the mesencephalic pretectal nucleus or the rostral colliculus (see Fig. 2-5 through 2-7). The pretectal nucleus functions in the pupillary light reflex pathway. Optic tract axons terminate in this nucleus. Most of the axons of the neuronal cell bodies in the pretectal nuclei cross in the caudal commissure to synapse in the contralateral general visceral efferent (GVE) parasympathetic oculomotor nucleus. Thus light entering one eye will have its greatest effect on the pupil of that eye because of the crossing of the majority of the axons in the optic chiasm and again at the caudal commissure.
For somatic motor responses to retinal activity, the axons that course into the rostral colliculus form the brachium of the rostral colliculus, which lies between the lateral geniculate nucleus and the rostral colliculus. The rostral colliculus is also a laminated structure, and, in addition to the optic tract axons, it receives axons from the cerebral cortex (especially the visual cortex) and the spinal cord via the spinomesencephalic (spinotectal) tract in the ventral funiculus. Recall from Chapter 3 that the tectum of the mesencephalon is the roof composed of the four colliculi. Tectonuclear axons of cell bodies in the rostral colliculus project to the tegmentum of the midbrain and medulla to influence the nuclei of cranial nerves III, IV, and VI. A tectospinal tract courses caudally from the rostral colliculus, decussates in the midbrain, and continues through the medulla into the ventral funiculus of the cervical spinal cord to contribute to the upper motor neuron that influences the lower motor neurons in the gray matter of the cervical spinal cord.153 These rostral collicular efferent tracts are important in the activation of muscles concerned with the orientation of the eyes, head, and neck in response to visual input. Think of the muscles that are activated for you to follow the action in a sports event or in your response to a flashbulb. Tectothalamic axons project to the thalamus for feedback to the cerebral cortex, and tectocerebellar axons project to the cerebellum via the rostral cerebellar peduncle. All of these pathways function in the coordination of head, neck, and eyeball movements in response to visual stimuli.
CLINICAL EVALUATION
Clinical Tests
Vision can be tested by watching the patient walk in a strange environment or through a maze of obstacles. Owners may be unaware of a loss of vision in their pet if its activity is confined to their home or yard and especially if no stairs are available to negotiate. Owners rarely detect unilateral visual loss. In the neurologic examination, vision is evaluated by the menace response and pupillary light reflexes. 44,46
Menace Response Test
The menace response test consists of making a menacing gesture with the hand directed at one eye while the other eye is covered and observing closure of the eyelids. You can perform this test as you face your patient or while standing over your patient facing in the same direction. Care must be taken not to touch any of the facial hairs or to create too much air turbulence because a palpebral reflex will be elicited. Cover one eye with your hand that is holding the head and lightly tap the eyelids or the side of the face of the eye being tested so that the patient is aware of the test and to be sure the facial nerve functions normally. The menace response is a learned response that requires the entire peripheral and central components of the visual system pathway and connections from the cerebrum to the brainstem with activation of the facial neurons in the medulla. Being a learned response, it is often absent in young animals. It is, however, usually present in foals and calves by 1 to 2 weeks of age and by 10 to 12 weeks of age in puppies and kittens. In patients with facial nerve paralysis, look for retraction of the eyeball away from the menacing gesture, which will be indicated by a brief protrusion of the third eyelid. Occasionally, this patient will retract its entire head away from the gesture. Based on the observation of numerous kinds of lesions located in the cerebral components of this visual pathway, it has been established that these areas are all necessary for the perception of vision required for this menace response. Experimental studies have defined a role for the rostral colliculus in the normal menace response, but the rarity of lesions localized to one or both rostral colliculi does not permit any similar clinical conclusion.
In all domestic animals, diffuse cerebellar disorders, especially those that involve the interposital and lateral cerebellar nuclei, have often been observed to cause a failure of the menace response to elicit eyelid closure bilaterally. The palpebral reflex is normal, as is their vision, based on eyeball or head retraction. The assumption is either that the pathway that mediates this response passes through the cerebellum to reach the facial neurons (Fig. 14-14) or that the cerebellar lesion causes inhibition of the cerebrocortical neurons involved in this response. An anatomic pathway was described in Chapter 13 on the cerebellum that involves a synapse in the pontine nuclei, a cerebropontocerebellar pathway. Alternatively a pathway may involve the rostral colliculus with or without a pontine relay, a cerebrotectopontocerebellar pathway115 or cerebrotectocerebellar pathway. See Video 13-22 and Video 13-23 in Chapter 13 for a good example of this feature in Arabian foals with a diffuse cerebellar cortical abiotrophy. In the horse and dog, we have observed a unilateral loss of the menace response in patients with a large unilateral cerebellar lesion on the same side as the menace deficit. This loss may be explained by the crossing that occurs from the cerebrum to the cerebellum at the pontine nucleus (see Fig. 14-14).
Pupillary Light Reflex
The pupillary light reflex was described in Chapter 7. Fig. 7-1 highlights this pathway. The cones are probably the most receptive to this bright light stimulus. Light should be directed toward the lateral (temporal) retina, where the area centralis is located. This procedure tests the visual components of the eyeball and the central visual pathway to the level of the mesencephalic pretectal nuclei at the junction of the thalamus and midbrain. The light reflex pathway continues to the adjacent GVE parasympathetic preganglionic neurons in the rostral portion of the oculomotor nuclei, which mediate the motor response. No involvement of any component of the cerebrum occurs in this reflex. Recall that the direct response to light directed into one eye is greater than the indirect (consensual) response in the other eye because of the majority of optic nerve axons crossing in the optic chiasm and the majority of the pretectal nuclear axons crossing through the caudal commissure. This feature is more pronounced in horses and farm animals than in small animals. Lesions in the afferent (visual) portion of this pathway must be severe to cause a loss of this reflex. Remember that the rate of pupil constriction varies among species of domestic animal, being most rapid in cats and slowest in horses. Occasionally, when this reflex is tested, the bright light causes the eyelids to close. This reaction is called the dazzle reflex, or squint, and involves optic tract axons that synapse in the rostral colliculus and tectonuclear axons that synapse in the facial nucleus to elicit this motor response. This route is a brainstem pathway similar to the pathway for constriction of the pupils and does not involve the cerebrum.
Clinical Signs
See Table 14-1. Refer to Fig. 7-1 to help you determine what to expect with the following location of lesions. The degree of visual deficit depends on the location and extent of the lesion in the visual pathway.
All domestic animals with these unilateral lesions in the central visual pathway exhibit a poor to absent palpebral closure response to menacing gestures to the eyeball that is contralateral to the lesion. If the examiner is careful, this test can be reliably performed without using a transparent barrier between the hand and the eyeball to prevent air currents and direct contact with facial hairs. The dropping of cotton balls, which some recommend instead of the menace test, is unreliable and awkward to perform. In small animals, the eye not being tested must be covered. By menacing the eye from both the medial and lateral visual fields when a lesion exists in the contralateral central visual pathway, you may determine that the deficit is more pronounced when the lateral visual field is tested.
In unilateral lesions of the central visual pathway caudal to the optic chiasm, the pupillary light reflex responses are usually normal. The logical assumption is that a unilateral lesion in the optic tract will cause a decreased pupillary light reflex in the contralateral eyeball because most of the optic nerve axons cross in the optic chiasm and most of the axons of pretectal neurons cross back to the oculomotor nucleus on the side of the eyeball that is stimulated (see Fig. 7-1). In our experience, this difference in the pupillary light reflex response between the two eyeballs with a unilateral optic tract lesion has been difficult to appreciate.
Signalment: 3-year-old male Doberman pinscher
Chief Complaint: Widely dilated left pupil
History: For at least the past 6 days, the left pupil was noticed to be wider than the right pupil.
No ancillary studies were performed, and 1 week later, this patient was examined again because of progressive depression. This dog was now obtunded and reluctant to stand. If stimulated, he could stand and walk with a normal but slow gait. Postural reactions were slow bilaterally. The cranial nerve examination was unchanged. These observations supported involvement of the ascending reticular activating system (ARAS) in the rostral brainstem. The severity of the obtundation in a dog that could still walk indicated involvement of the ARAS in the diencephalon. At this level, the oculomotor nerve is ventral to the hypothalamus. With the initial clinical signs limited to the left oculomotor nerve, an extraparenchymal (extraaxial, extramedullary) lesion was presumed, and a mass lesion that compressed this nerve and subsequently the hypothalamus was the presumptive clinical diagnosis. At this age, a germ-cell neoplasm is more common than a pituitary neoplasm or a meningioma. Most pituitary neoplasms grow dorsally into the hypothalamus and do not usually involve the cranial nerves passing through the middle cranial fossa to enter the orbital fissure. They also usually spare the optic nerves and chiasm. This dog was euthanized, and a large germ-cell neoplasm was found at necropsy (Fig. 14-15). In humans, the GVE preganglionic neurons in the oculomotor nerve are on the surface of the nerve where it courses through the middle cranial fossa. Thus these neurons are more at risk for compressive lesions, and pupillary light reflex abnormalities usually precede clinical signs of involvement of the GSE lower motor neurons in this cranial nerve. This neoplasm is readily demonstrated on magnetic resonance (MR) imaging (Fig. 14-16).
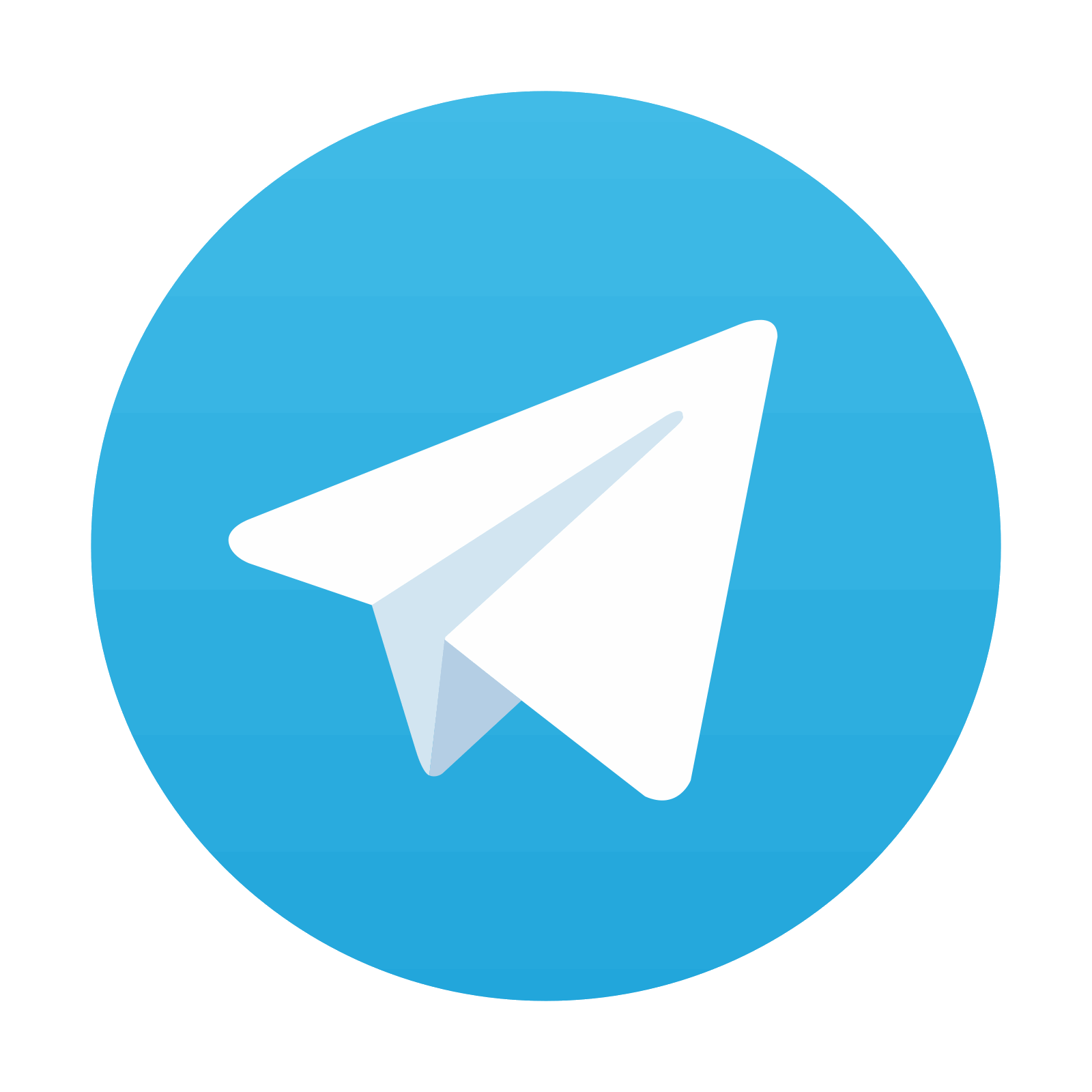
Stay updated, free articles. Join our Telegram channel
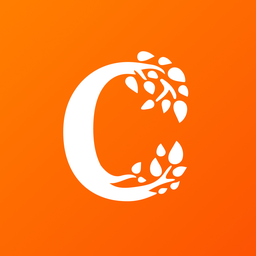
Full access? Get Clinical Tree
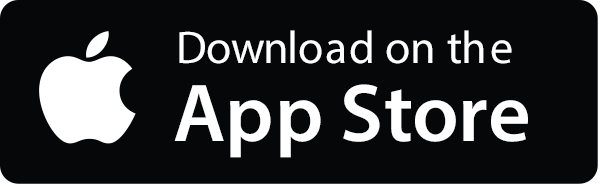
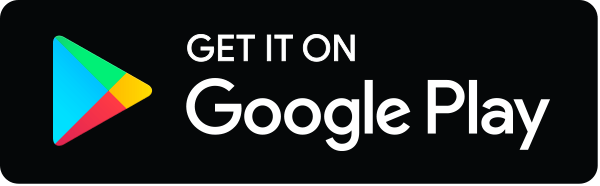