CHAPTER 4 Cerebrospinal Fluid and Hydrocephalus
PRODUCTION
The CSF originates from capillaries throughout the CNS and leptomeninges. A major site is the choroid plexus located in the lateral, third, and fourth ventricles. Small amounts enter the ventricles from the parenchyma through their ependymal lining and enter the subarachnoid space through the pial-glial membrane on the external surface of the parenchyma. Capillaries within the parenchyma are a small source via the microscopic interstitial spaces. Leptomeningeal capillaries in the subarachnoid space are an additional small source of CSF. One study of CSF production determined that 35% was derived from the third and lateral ventricles, 23% from the fourth ventricle, and 42% from the subarachnoid space.3,4,8,15
The choroid plexus consists of numerous fronds of villous processes that project into the CSF of the ventricular system. The simple cuboidal cells of the plexus are continuous with the ependymal cells that line the ventricular surface adjacent to the CNS parenchyma (Fig. 4-1).19 At the choroid plexus, there are essentially two cell layers between the blood plasma and the ventricular CSF. The vascular endothelium is separated from the choroidal epithelial cells by a thin basement membrane and loosely arranged meningeal pial cells. The endothelial cells of the choroid plexus capillaries are considered to be fenestrated because they lack tight junctions. This is different from the capillary structure within the parenchyma. The epithelial cells of the choroid plexus are robust cuboidal cells with tight junctions at their ventricular surfaces. They have the characteristics of cells that function in the transcellular transport of materials. This includes microvilli on their luminal surface and infoldings of the basal portion of the cell membrane. This is a semipermeable barrier that selectively and actively transports some substances and inhibits others. The methods of CSF production are a selective ultrafiltrate from the blood plasma and active transport mechanisms that utilize energy.
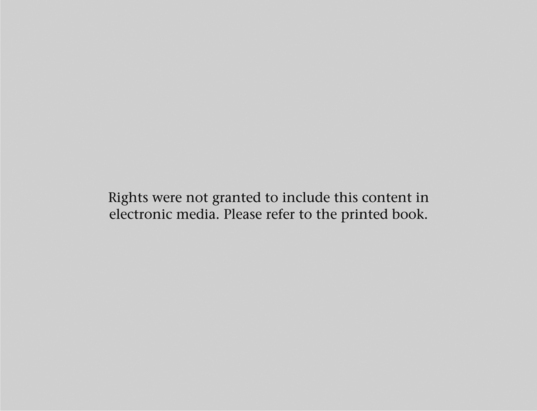
Rights were not granted to include this table in electronic media. Please refer to the printed book.
From Strazille N, Ghersi-Egea S-F: Choroid plexus in the central nervous system: biology and physiopathology, J Neuropathol Exp Neurol 59:561-574, 2000.
The rate of production of CSF varies with the species and the method of determination but is remarkably rapid. The total volume of CSF is produced and absorbed about 3 to 5 times per day. The following rates have been determined: dog, 0.047 ml/min; cat, 0.017 ml/min. With this continuous turnover of CSF, there is evidence that it is produced at a constant flow rate, regardless of increases or decreases in CSF pressure in the ventricular system. In chronic obstructive hydrocephalus, atrophy of the choroid plexus decreases CSF production. The rate of CSF production is independent of the hydrostatic pressure of the blood but is influenced by the osmotic pressure of blood. Intravenous administration of hypertonic solutions reduces the rate of formation of CSF. This has clinical application in head injuries involving brain edema and in neurosurgery performed for space-occupying lesions. An osmotic diuretic is administered to decrease the rate of CSF formation and to decrease the vasogenic edema associated with the brain lesion. Brain volume will be reduced and intracranial pressure will be lowered. The most common osmotic agent used is mannitol, a hypertonic solution of a carbohydrate. It is administered intravenously at 0.25 to 2 g/kg of a 20% solution. If mannitol is administered intraoperatively, the reduction in the size of the brain is grossly visible.
MENINGES: SUBARACHNOID SPACE
Before we consider the circulation of CSF, it is worthwhile to review the anatomy of the meninges, which is described with the dissection of the brain and spinal cord in Guide to the Dissection of the Dog (ed 6, by H. E. Evans and A. de Lahunta). Recall that the entire CNS is covered by three layers of connective tissue (Fig. 4-2). These layers are derived predominantly from the neural crest with a small component of mesoderm. The most external layer is the thick, fibrous dura mater that, within the cranial cavity, closely adheres to the bones of the skull. Within the vertebral canal, the dura is separated from the vertebrae by the epidural space, which often contains fat. The arachnoid membrane is a thin layer of connective tissue that in life adheres to the dura by means of desmosomal attachments (Fig. 4-3).10 There is no subdural space in the normal living animal, despite what you may read in the literature. These dura-arachnoid desmosomal attachments are easily disrupted by hemorrhage following an injury (subdural hematoma); by contrast agents inadvertently injected into the dura-arachnoid; and following death, when the CSF pressure is lost. It should be noted here that subdural hematomas are rare in veterinary medicine compared with their occurrence in humans. This arachnoid membrane is the external surface of the subarachnoid space, which contains CSF, blood vessels, spinal nerve roots, and arachnoid trabeculations. The latter are thin, collagenous extensions from the arachnoid membrane to the pia mater, which is the thin layer of connective tissue attached to the surface of the CNS parenchyma where it forms a pial-glial membrane. The thick dura mater is also called the pachymeninx, and the thin vascularized pia and arachnoid make up the leptomeninges.
CIRCULATION
The CSF circulates from the ventricular system to the subarachnoid space by way of the lateral apertures of the fourth ventricle (Fig. 4-4). In some animals there is a similar passage between the central canal and the subarachnoid space at the conus medullaris (see Fig. 4-2). Primates also have a median plane aperture (the foramen of Magendie) in the caudal part of the roof plate of the fourth ventricle. Much of the CSF passes dorsally over the cerebellum, ventral to the tentorium, and then over the cerebrum where it has access to the venous sinuses. The CSF covers the entire external surface of the brain and spinal cord, where it can penetrate the parenchyma along with the larger blood vessels in their perivascular spaces. These spaces are extensions of the subarachnoid space to the point where the pia mater blends with the adventitia of the blood vessel. This is not a distinct point, and for some distance the cells of the leptomeninges and the adventitia of the blood vessel may be closely related and the perivascular space reduced to small clefts between cells. Ultimately the CSF has access to the very small extracellular interstitial spaces of the CNS parenchyma. Altered CSF flow patterns along the spinal cord subarachnoid space lead to syrinx formation when CSF enters the spinal cord parenchyma along these perivascular spaces. These flow pattern alterations may also contribute to the formation of a subarachnoid diverticulum. These diverticula are incorrectly referred to as subarachnoid cysts in the literature.
The flow of CSF in the ventricles is thought to be caused primarily by the pulsations of blood in the choroid plexuses. Remember that the cranial cavity is a closed space consisting of three components: the brain parenchyma, the blood, and the CSF (Fig. 4-5). Any increase in the volume of one of these compartments must be compensated for by a reduction in the volume of another compartment. With each arterial pulsation, the CSF pressure rises and surges toward the lateral apertures. The cilia on the ependymal cells may make a small contribution to this flow. Movement of the CSF within the subarachnoid space of the cranial cavity and from the cranial cavity to the spinal cord subarachnoid space is also dependent on cardiac systole and intracranial arterial pulsations in that closed space. Interference with this flow at the foramen magnum can lead to the development of a syringohydromyelia, as discussed in the section about brain malformations in Chapter 3. The effect of this arterial pulsation on CSF movement is remarkable when viewed using magnetic resonance (MR) imaging utilizing CSF flow analysis (see Video 4-1). In addition, during surgery that involves the ventricular system or subarachnoid space, the surgeon can directly view the arterial pulsatile effects on CSF flow.
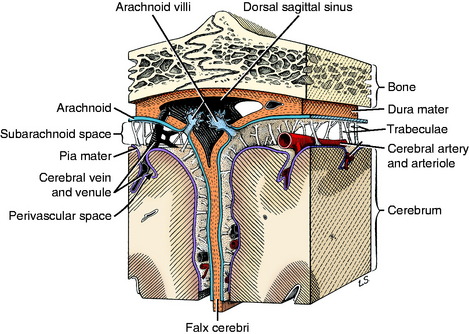
Figure 4-5 Cerebral meninges and arachnoid villi at the attachment of the falx cerebri to the calvaria.
In relationship to cardiac systole and diastole, the CSF can move cranially and caudally, but the caudal flow predominates. Radioiodinated serum albumin injected into the lumbar cistern of humans can be followed by scanning radiographic procedures to the dorsum of the cerebrum in 12 to 24 hours. Similar injections into the lateral ventricle appear in the thoracolumbar subarachnoid space in 30 to 40 minutes, reflecting the predominant caudal flow of CSF.6 Comparison of cerebellomedullary and lumbosacral CSF in animals with focal spinal cord disease supports the predominance of the caudal flow of CSF.
When the intracranial space is compromised by developing lesions, the CNS is able to accommodate for it by physiologic mechanisms that are referred to as autoregulation. The purpose of this autoregulation is to attempt to maintain adequate cerebral perfusion in the presence of increased intracranial pressure caused by the lesions. This perfusion is independent of systemic blood pressure. Cerebral perfusion pressure is defined as the mean arterial blood pressure minus the intracranial pressure. It determines the oxygen and nutritional supply to the parenchyma. This autoregulation involves complex regulation of intracranial blood flow by chemical, neurogenic, and myogenic means. It is most effective with slow-growing lesions that occupy space. It is amazing how large neoplasms can be that develop within the prosencephalon or even in the extraparenchymal (extraaxial) space along the brainstem without producing neurologic signs as long as their growth is slow. Slowly growing extramedullary lesions can cause more than a 50% compression of the spinal cord before clinical signs are seen. CNS vascular autoregulation is the basis for the preservation of the parenchymal perfusion to these compromised tissues. Clinical signs occur when the tissue demands exceed the capability of this autoregulation. Vasogenic and cytotoxic edema follow. When this occurs within the cranial cavity, the uncontrolled increase in intracranial volume and pressure forces displacements of various components of the brain that are referred to as herniations. These represent attempts of the brain to squeeze out of the cranial cavity. Three of these herniations are regularly seen in domestic animals. The subfalcine herniation is a medial displacement of one cerebral hemisphere ventral to the falx cerebri. On computed tomography (CT) and MR imaging, a sign commonly seen is referred to as a midline shift; it represents an enlargement of one cerebrum (Figs. 4-6 and 4-7). When it is severe, a subfalcine herniation may occur. The portion of the enlarging cerebral hemisphere that is herniated is the cingulate gyrus. The transtentorial herniation is the displacement of the caudal aspect of one or both cerebral hemispheres ventral to the tentorium cerebelli. This directly compresses the mesencephalon and indirectly the rostral cerebellum. It can sometimes be seen on MR imaging but is recognized at necropsy by the marked oblique indentation in the caudomedial aspect of the occipital lobes and often includes the parahippocampal gyrus on the medial aspect of the cerebral hemisphere (Figs. 4-8 and 4-9). Herniation of the caudoventral aspect of the cerebellar vermis is commonly called cerebellar coning. This involves primarily the pyramis and uvula lobules, which compress the ventrally situated medulla. It can sometimes be seen on axial (transverse) MR images but is best seen on median-plane sagittal MR images (Figs. 4-10 and 4-11). It should not be referred to as “tonsillar” herniation because these paramedian lobules do not herniate in domestic animals, only in primates.
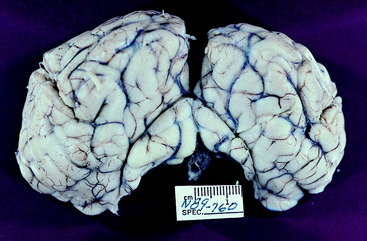
Figure 4-8 Gross lesion of the occipital lobes where a transtentorial herniation occurred. This is the cerebrum of the 14-year-old Morgan horse with the cholesterinic granuloma of the choroid plexus of the left lateral ventricle seen in Fig. 3-19. The herniation is worse on the left side. Herniation on the right side is due to the vasogenic edema crossing through the corpus callosum into the white matter of the right cerebral hemisphere.
ABSORPTION
The major site of CSF absorption is at the arachnoid villi that are located in the venous sinuses or cerebral veins.20 Collections of these arachnoid villi are known as arachnoid granulations. Occasionally they can be seen in some species on gross examination of the lumen of a large intracranial venous sinus.8,9 The arachnoid villus is a prolongation of the arachnoid membrane and subarachnoid space into the lumen of the venous sinus covered by the endothelium of the vessel wall (see Fig. 4-5). Usually the portion of the villus within the lumen is reduced to a single endothelial cell between the CSF of the subarachnoid space and the lumen of the sinus. It is structured to act as a one-way valve that permits the CSF to flow into the lumen of the sinus when the CSF pressure exceeds venous pressure. When the intravenous pressure exceeds the CSF pressure, these villi collapse, closing the “valves” so that no blood can pass into the subarachnoid space. Flow is one-way at these sinuses, only from the CSF into the blood.17 Electron microscope studies of these endothelial cells that line the arachnoid villi have revealed transient transcellular channels that develop for the passage of materials from the CSF into the venous system.1 These apparently develop in response to a pressure gradient between the CSF and venous blood.
Other sites of CSF absorption include the veins and lymphatics found around the spinal nerve roots and spinal nerves at the intervertebral foramina and associated with the first, second, and eighth cranial nerves where they pass through the skull bones. CSF passes through the cribriform lamina of the ethmoid bone, where it is absorbed or leaks into the nasal cavity, resulting in cerebrospinal rhinorrhea.7 The small amount of CSF in the interstitial spaces of the parenchyma may be absorbed into the parenchymal blood vessels.
FLUID COMPARTMENTS
The blood-CSF barrier exists between the plasma and the CSF at the choroid plexus and consists of two cell layers separated by a thin basement membrane. The vascular endothelial layer and its basement membrane have fenestrations, and the cells lack tight junctions. The choroidal epithelium consists of large cuboidal cells with tight junctions at their ventricular surfaces. Fragments of meningeal cells are interspersed among these endothelial and epithelial cells (see Fig. 4-1). They serve as a semipermeable membrane between the plasma and the CSF. In acid-base imbalances, CO2 readily passes between the plasma and the CSF, whereas bicarbonate exchange is slow owing to the relative impermeability of this barrier to bicarbonate.
FUNCTION
CSF serves to protect the CNS by its physical support, with the parenchyma suspended in this fluid medium. It protects the CNS by its role in modulating pressure changes that occur, especially in the closed cranial cavity. In conjunction with cerebral blood flow, the CSF helps to regulate the intracranial pressure. It protects the parenchyma by being a source of nourishment because it is a medium for the transport of metabolites and nutrients between the blood and the CNS parenchyma. It also serves to transport neuroendocrines and neurotransmitters within the parenchyma. CSF plays a role in maintaining the ionic balance necessary for neuronal function by acting as a chemical buffer for the parenchyma. By means of its close relationship to the extracellular fluid in the interstitial spaces, the CSF provides a more stable and closely controlled ionic environment than the blood plasma. At necropsy, when studying young animals with extensive leptomeningitis, it is surprising how profound their diffuse neurologic signs are with these lesions confined to the subarachnoid space in the absence of any microscopic parenchymal lesions. Cryptococcal meningitis in dogs and cats and bacterial meningitis in calves are examples of this observation, which reflects the importance of the CSF in providing vital nourishment to maintain parenchymal function.
CEREBELLOMEDULLARY CISTERN CSF COLLECTION
For most dogs and cats, a spinal needle with a stylet that is 1.5 inches long and 20 or 22 gauge is adequate. A 3.5-inch, 20- or 22-gauge spinal needle may be necessary in some of the larger dog breeds. In the horse and ox, a 3.5-inch, 18- or 20-gauge spinal needle may be used (Fig. 4-12). With the bevel directed to one side, insert the needle through the skin and underlying fascia and muscle, directing it toward the angle of the mandible. To support the needle for removing the stylet, place the palm of your left hand on the head over the external occipital protuberance for support. Grasp the hub of the needle with the fingers of your left hand and do not move your left hand until the procedure is completed. Remove the stylet with your right hand and observe for fluid. You want to know how far to go! Well, we can not tell you because there is so much difference between a Chihuahua and a Great Dane. So use trial and error carefully. We recommend that you initially practice this on a few euthanized patients. Insert the needle progressively and observe for CSF at the hub at 1-mm intervals. Occasionally you will feel a slight loss of resistance when you penetrate the atlantooccipital membrane and dura together and enter the subarachnoid space, but do not count on that feeling. Because the cistern is small in all cats and the small breeds of dogs, holding the needle hub tightly, with the left hand braced on the head, will prevent you from dislodging the needle when you remove the stylet. If you strike bone, you may be able to judge which bone you are on and walk the needle either cranially off the atlas or caudally off the occipital bone before inserting the needle farther. Usually when you are in the cistern, the CSF will immediately appear in the needle hub. Do not advance the needle without the stylet so that tissue will not obstruct the needle. In small patients, such as cats and toy-breed dogs, use a 21-gauge butterfly needle because the capillary forces will contribute to the flow of CSF.
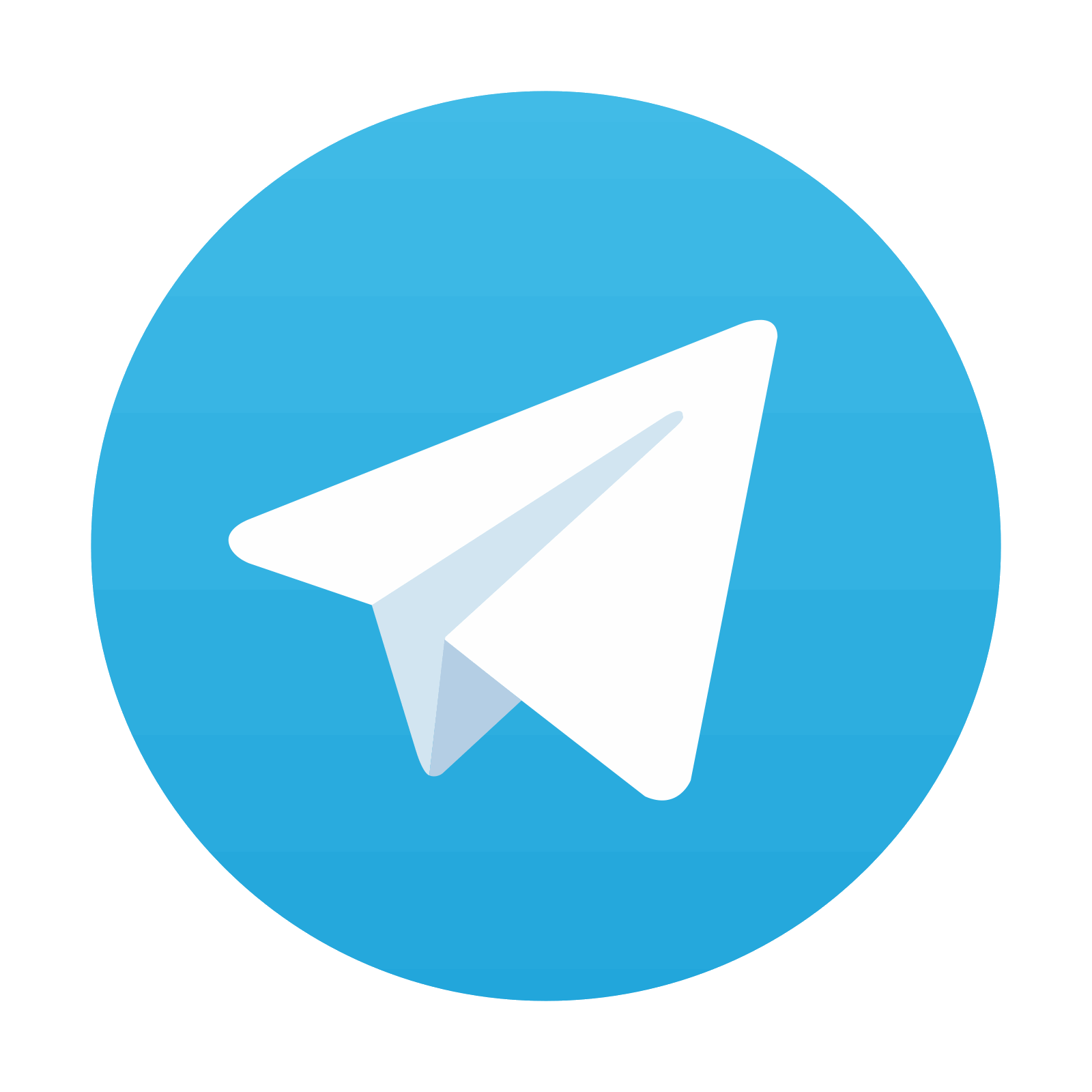
Stay updated, free articles. Join our Telegram channel
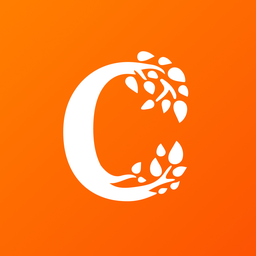
Full access? Get Clinical Tree
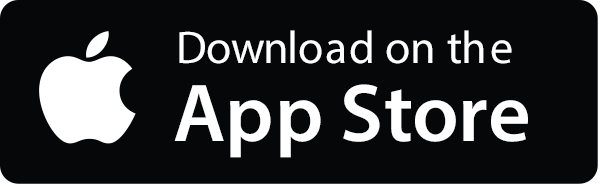
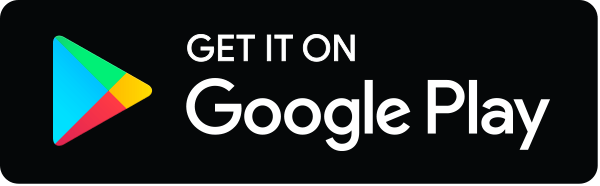