Chapter 9 Treatment of Fungal Infections
Fungal Physiology
The pathogenic fungi affecting humans and animals are eukaryotes, generally existing as either filamentous molds (hyphal forms) or intracellular yeasts (Table 9-1).1 Dimorphic fungi grow in the host as a yeastlike form but as molds in vitro at room temperature. Some fungi (e.g., Coccidioides immitis, Histoplasma, and Rhinosporidium species) grow inside host cells, dividing into spores until released from the cell as it ruptures. The fungal cell wall is a target for several of the antifungal drugs (Figure 9-1). It is rigid and contains chitin, a structural component, and polysaccharides. These generally preclude Gram staining and serve as a barrier to drug penetration. The cell membrane is complex and, unlike bacteria but as with higher eukaryotes, contains sterols.2 Ergosterol is the primary sterol component of fungal cell membranes, regulating both permeability and membrane-bound enzymes. Because it also is a major component of organelle membranes, it also influences mitochondrial respiration and oxidative phosphorylation.3 The rate of chitin synthesis is influenced by ergosterol content, being inhibited at high concentrations and stimulated at low concentrations. Fungal content of ergosterol influences drug efficacy and the potential risk of resistance for some drugs. Microtubular structures found in all eukaryotic cells support the cellular cytoskeleton and mitotic spindle; they support not only cell division but also cellular integrity. Organelle position and movement are supported by microtubules. The tubules in turn are comprised of tubulin, a heterodimer containing α and β subunits. Because microtubules are constantly being assembled and dissembled, impaired microtubular synthesis alters integral cellular function.
Table 9-1 Classification of Selected Fungi of Medical Importance
Level | Name | Disease or Infecting Organism |
---|---|---|
Kingdom | Fungi | |
Subkingdom | Amastigomycotera | |
Phylum | Zygomycota | |
Class | Zygomycetes | |
Order | Mucorales | |
Family | Mucoraceae | |
Genus | Absidia | Absidia (Zygomycosis, mucormycosis) |
Mucor | Mucor (Zygomycosis, mucormycosis) | |
Rhizopus | Rhizopus (Zygomycosis, mucormycosis) | |
Family | Several others | |
Order | Entomophthorales | |
Family | Basidiobolaceae | |
Genus | Basidiobolus | Basidiobolus sp. |
Subkingdom | Eumycotera | |
Phylum | Ascomyta | |
Subphylum | Saccharomycotina | |
Order | Saccharomycetales | |
Family | Saccharomycetaceae | Budding yeasts |
Genus | Saccharomyces | Budding yeasts |
Genus | Debaryomyces | Candida∗ |
Khyveromyces | Candida∗ | |
Lodderomyces | Candida∗ | |
Pichia | Candida∗ | |
Subphylum | Pneumocystiodiomycetes | |
Order | Pneumocystidiales | |
Family | Pneumocystideaceae | |
Genus | Pneumocystis jirovecii | Pneumocystis pneumonia |
Subphylum | Euascomycotina | |
Order | Eurotiales | |
Family | Eurotiaceae | |
Genus | Eurotium, Emericella | Aspergillus∗ |
Genus | Talaromyces | Penicillium∗ |
Order | Onygenales | |
Family | Gymnoascaceae/Ajellomycetaceae | |
Genus | Ajellomyces | Blastomyces dermatitidis∗ |
Histoplasma capsulatum∗ | ||
Paracoccidioides braziliensis∗ | ||
Family | Gymnoascaceae/Arthrodermataceae | |
Genus | Arthroderma | Microsporum |
Trichophyton | ||
Epidermophyton | ||
Class | Pyrenomycetes/Sordariomycetes | |
Order | Ophiostomatales | |
Family | Ophiostomataceae | |
Genus | Ophiostoma | Sporothrix schenckii∗ |
Order | Hypocreales | |
Family | Many | Fusarium |
Order | Clavicipitales | |
Family | Clavicipitaceae | |
Genus | Ergot alkaloids | St. Anthony’s fire |
Phylum | Basidiomycota | |
Subphylum | Holobasidiomycont Basidiomycot | |
Class | Phragmobasidiomycetes/Tremellomycetes | |
Order | Trichosporonales | Trichosporon asahii∗ |
Order | Filobasidiales | |
Family | Filobasidiaceae | |
Genus | Filobasidiella | Cryptococcus neoformans∗ |
Phylum | Fungi Imperfecti/Deuteromycota | |
Form–Class | Blastomycetes | |
Form–Order | Cryptococcales | |
Form–Family | Cryptococcaceae | |
Genus | Candida | Candida |
Genus | Cryptococcus | Cryptococcus |
Genus | Malassezia | Malassezia |
Genus | Pityrosporum | Pityrosporum |
Genus | Rhodotorula | Rhodotorula |
Genus | Trichosporon | Trichosporon |
Form–Class | Hyphomycetes | |
Form–Order | Moniliales | |
Form–Family | Moniliaceae | |
Genus | Aspergillus | Aspergillus |
Genus | Blastomyces | Blastomyces |
Genus | Coccidioides | Coccidioides |
Genus | Epidermophyton | Epidermophyton |
Genus | Geotrichum | Geotrichum |
Genus | Hostoplasma | Hostoplasma |
Genus | Microsporum | Microsporum |
Genus | Paracoccidioides | Paracoccidioides |
Genus | Penicillium | Penicillium |
Genus | Sporothrix | Sporothrix |
Genus | Trichophyton | Trichophyton |
Genus | Others | |
Form–Family | Dematiaceae | Alternaria |
Genus | Alternaria | Bipolaris |
Genus | Bipolaris | Cladophialophora |
Genus | Cladophialophora | Curvularia |
Genus | Curvularia | Helminthosporium |
∗ Teleomorphic form or sexually producing state.
(From Reference Guide to the Classification of Fungi and Fungal-like Protists, with Emphasis on the Genera with Medical Importance (circa 2007), accessed December 17, 2009, at http://www.sbs.utexas.edu/mycology/bio329/pdf_files/sp2007/refguidefungal_sp2007.pdf.)
Pathophysiology of Infection
Fungal infections differ from bacterial infections in several respects, and pathogenic fungi have developed several characteristics that complicate antimicrobial therapy.4 Some of the differences in the eukaryotic structure of fungal organisms compared with the prokaryotic structures of bacteria were delineated in the preceding section. Other differences exist. For example, Cryptococcus and occasionally Sporothrix schenkii produce an external coating or slime layer that encapsulates the cells and causes them to adhere and clump together.2 Whereas many fungal organisms produce exotoxins in vivo, there is no conclusive evidence that fungi produce endotoxins. Fungal organisms are characterized by a low invasiveness and virulence. In fact, most animals will overcome a fungal infection. Factors that predispose the patient to infection include necrotic tissue, a moist environment, and immunosuppression. Innate immunity generally rapidly identifies and destroys fungal organisms that present pathogen-associated molecular patterns recognized by specific receptors on host cells. Pattern recognition receptors include the lectinlike receptor dectin-1, which binds beta-glucan in the fungal cell wall, and the Toll-like receptors. Binding by neutrophils and macrophages results in a cascade of intracellular events that cause rapid clearance in tissues of exposure. Organisms that are able to penetrate these tissues initiate an immune response. Immunity to fungal organisms appears to be T-cell mediated, with tumor necrosis factor-alpha (TNF-α) being a major signaling cytokine, although all but dermatophytes also stimulate antibody production.5 In response, fungal organisms impair an effective immune response through a number of mechanisms. A number of molecules scavenge oxygen radicals generated by inflammatory cells (melanin, mannitol, catalase). The cell wall of Histoplasma capsulatum contains β-glucan, which covers and hides α-glucan, preventing recognition by phagocytic cells. Further, it is able to survive intracellular locations in phagocytic cells by a number of mechanisms. Phenotype switching prevents receptor recognition by host cells.5 Gliotoxin is an immunosuppressive mycotoxin, produced particularly well by Aspergillus fumigatus.5
Although an adequate immune response and recruitment of appropriate inflammatory cells to the site of infection is critical to resolution of fungal infections, progression to uncontrolled, pyogranulomatous inflammation is often deleterious. Proper early cellular recruitment may determine the difference between success and failure. The positive effects of some antifungals may include their immunomodulatory capabilities. These beneficial effects, which are largely based on in vitro studies, with an occasional murine mouse model verification, have been reviewed by Ben-Ami and colleagues.5 Amphotericin B, the azoles, and the echinocandins have been studied most. Among the effects is downregulation of inflammatory cytokine genes. The use of antiinflammatories should be considered in critical situation. The use of glucocorticoids is addressed in the section on the treatment of fungal infections, but other antiinflammatories that minimally affect lymphocytic-mediated immunity (e.g., nonsteroidal antiinflammatories, leukotriene receptor antagonists) should be considered as long as attention is paid to potential negative drug interactions involving imidazoles in particular. Manipulation of the immune response in combination with antifungal drugs is a more recent area of focus that is likely to affect treatment of fungal disorders.
Fungal infections can be primarily superficial and irritating (e.g., dermatophytosis) or systemic and life threatening (e.g., dimorphic fungal infections including blastomycosis, cryptococcosis, histoplasmosis, and coccidioidomycosis). Fungal organisms may exhibit an affinity for certain tissues, such as the dermatophytes for keratin and H. capsulatum for macrophages. Animals may develop a hypersensitivity to the infecting organism (as is often seen in dermatophyte infections), which can result in a pathologic response to the infection as well as facilitate dissemination. The role of proteolytic enzymes in infections caused by dermatophytes is being investigated. On the other hand, the lack of hypersensitivity may also indicate a poorer prognosis for recovery.2
Information regarding antifungal drug use in animals is limited. Human literature often focuses on candidiasis, an infection that is much less common in dogs and cats; as such, relevance of information must be considered. The risk factors for fungal infection have been recently reviewed.7 Using candidiasis as an example, broad-spectrum antimicrobials or immunosuppressive drugs (glucocorticoids, chemotherapy) play a major role. Malnutrition, malignancy, age extremes, and neutropenia also predispose to candidiasis. Similar risk factors occur for aspergillosis, although increased organ transplantation and its accompanying immunosuppression probably has contributed to not only the increased incidence of infection but also the emergence of newer species. Likewise, the increased invasiveness of life-extending procedures coupled with increased survival is contributing to an increased incidence of infection. Improved diagnostic techniques are allowing identification of previously unknown organisms; for example, other filamentous organisms that are emerging in human medicine include the Zygometes (Mucor, Rhizopus, Rhizomucor, Absidia, and Cunninghamella), Fusarium, Paecilomyces, and Scedosporium.
Several factors can lead to therapeutic failure or relapse after antifungal therapy.4 Most antifungals are fungistatic in action, with clearance of infection largely dependent on host response.6 In humans relapsing infections are not uncommon for selected Trichophyton species and for invasive mycoses in immunocompromised patients. In the latter group, aspergillosis infections are particularly problematic. As with bacteria, the pattern of fungal disease is constantly changing. The advent of acquired immunodeficiency syndrome in human patients has been important in the development of new strains of resistant organisms, and there remains a continuing need for development of new antifungal agents. However, this represents only one of many factors that increase the risk of fungal infection. In some instances, therapeutic failure reflects poor penetration of drug into infected tissues (particularly the central nervous system and bone) or into those organisms that are encapsulated.
Several organisms, particularly the superficial pathogens and systemic opportunistic organisms, have a primary resistance to antifungal drugs, contributing to therapeutic failure. Like antibacterial resistance, antifungal resistance can be intrinsic (primary) or acquired (secondary). A third type of resistance, referred to as clinical resistance, involves the progression or relapse that occurs despite laboratory-documented susceptibility to the treatment drug.8 Primary resistance occurs with amphotericin B to filamentous fungi and dermatophytes. The risk factors for acquired resistance have not been well identified. Because resistance is an increasingly emerging problem, dosing regimens should maximize antifungal plasma concentrations. However, much more so than antibacterial drugs, antifungal drugs present a risk of toxicity causing the design of dosing regimens to be much more restrictive compared to antibacterial therapy.
Toxicity of antifungals is a common cause of therapeutic failure. Because both the antifungal target organism and the host cells are eukaryotic, the cellular targets of fungal organisms are substantially different from those of bacterial organisms. As a result, antibacterials generally are ineffective against fungal organisms, and, in contrast to most antibacterials, antifungals are often toxic or associated with undesirable side effects in the host. The incidence of side effects has limited the number of effective yet safe antifungal drugs available. Some strategies for reducing toxicity have allowed dose escalation, increasing the likelihood of efficacy and decreasing the risk of resistance. Their use (e.g., liposomal amphotericin B products) are particularly important in the immunosuppressed patient. A potentially important strategy for avoidance of resistance is combination therapy.8,9 Combination therapy, if correctly designed, should also enhance efficacy and reduce toxicity through more rapid response to therapy. Dose reduction may be possible. Finally, a common reason for therapeutic failure is discontinuing therapy after resolution of clinical signs but before eradication of infection. Therefore antifungal therapy should extend well beyond clinical cure.
As with antibacterials, efficacy is influenced by the relationship between plasma or tissue concentrations, minimum inhibitory concentration (MIC) of the infecting microbe and antifungal. Studies for antifungal organisms generally begin with killing curves followed by animal models; however, as with antibacterials, their relevance also must be supported by pharmacokinetic studies that determine drug concentrations at the site of infection coupled with pharmacodynamic studies that determine susceptibility in terms of end points of efficacy.10
Compared with bacterial testing, antifungal culture and susceptibility testing have not been well developed as a tool for the treatment of fungal infections. As with antibacterial testing, antifungal testing is principally identification of resistant microbes rather than a description of the level of susceptibility.8 In vitro susceptibility testing of antifungal agents is highly dependent on test conditions, and interlaboratory results vary markedly. Interpretation of culture and susceptibility data may be limited by a lack of standardized testing methods for some drugs and organisms. As with bacteria, the MIC for a fungal organism is the concentration of the antifungal drug that inhibits the growth of the fungus under standardized conditions (Table 9-2). The minimum lethal concentration is the concentration that kills the organisms.1 Correlation between MIC and clinical response is poor, and assessment of antifungal agents appears to be best accomplished through efficacy studies in animal models. Fortunately, the need for fungal culture and susceptibility testing may not be as critical for fungal organisms as it is for bacterial organisms because, with the exception of 5–flucytosine, fungal development of resistance to antimicrobial therapy is not common.11 Resistance is more likely with a rapidly growing organism exposed to high concentrations of an antifungal for a long period of time.1 However, resistance does occur, and as with antibacterials, use of previous antifungal drugs appears to increase the risk of resistance. This has been demonstrated for Candida, the MICs of which tend to be higher in human patients previously treated with antifungals than in drug-naïve patients. Mechanisms of resistance of fungal organisms are similar to those of bacterial organisms (e.g., failure to accumulate in cells, altered target structures, formation of alternative pathways).12 The advent of newer antifungal agents and resistance among fungal organisms is, however, likely to cause in vitro testing of antifungals to become more important to therapeutic success.
One author notes that never before have so many new antifungals been under development, ranging from entirely new compounds with new targets to modifications of existing drugs (e.g., cyclodextrin–itraconazole and polyethylene glycol–amphotericin B).13 Newer concepts being explored include combinations of antifungals with one another or with nonantibiotic compounds. Newer therapies may focus on immunomodulation14 with a balance between recruitment (cytokines, chemokines, lymphokines, and growth factors) and antiinflammatory effects. The need for new therapies is timely, as the epidemiologic behavior of fungal organisms, at least as they occur in human medicine, increasingly is shifting toward opportunistic organisms for which traditional antifungals often are characterized by limited efficacy.7
Antifungal Drugs
The primary agents used to treat fungal infections are the natural antibiotics; the polyene macrolides (amphotericin B as the prototype); the synthetic agents, including the azoles (ketoconazole as the prototype); and the newer allylamine antifungals (Figure 9-2). Flucytosine has a less important role in the treatment of dimorphic fungal diseases, particularly in animals. The natural antibiotic griseofulvin belongs to no group but has an important place in the armamentarium against dermatophytosis. As recently as 1988, the treatment of systemic fungal infections in humans emphasized the use of amphotericin B, ketoconazole, and flucytosine. In the decade that followed, further development of the azole derivatives has led to a new age in the treatment of systemic fungal diseases. Currently, antifungal therapy is most effective when based on an understanding of the therapeutic ratio of the drug in the infection being treated. For amphotericin B, this ratio tends to be small because of its toxicity. The newer azole derivatives have proved to provide much of the efficacy of amphotericin B without its toxicity. Doses for selected antifungal drugs are found in Table 9-3.
Polyene Macrolide Antibiotics: Amphotericin B
Structure–Activity Relationship
Examples of polyene (i.e., multiple double-bond; see Figure 9-1) antifungal drugs include amphotericin B, nystatin, and pimaricin. Each antibiotic is produced by a different species of Streptomyces (family Actinomyces). Amphotericin B was developed in the 1960s and was so successful in fulfilling the need for a broad-spectrum antifungal that further advancement of antifungal therapy was largely ignored. These drugs are very large molecules, consisting of a macrolide containing a large lactone ring. The polyene contains three to eight double bonds, which represent the lipophilic portion of the molecule. The number of double bonds categorizes the polyenes into trienes, tetraenes (natamycin [paramycin]), and pentanes; amphotericin B and candicidin are heptanes, whereas nystatin is classified as a pseudo–heptane/tetraene.3 A hydroxylated hydrocarbon backbone represents the hydrophilic portion of the molecule. These compounds are insoluble in water and are unstable, and they will rapidly decompose if exposed to sunlight.
Mechanism of Action
Polyene macrolides bind with the sterol portion of the phospholipids that make up the fungal cell membrane. Amphotericin has a much higher affinity for ergosterol, the major sterol component of fungal cell membranes, than for cholesterol, the major sterol in mammalian cell membranes (Figure 9-3).1 The interaction of the drug and the sterol results in the formation of channels or pores in the cell membrane (Figure 9-4). The result is an increase in cell permeability and disruption in proton gradient flow; loss of membrane fluidity may alter H+-ATPase activity.3 Altered K+/H+ exchange results in the loss of K+ and Mg2+ from the cell. Cellular metabolism is disrupted; internal acidification of the fungal cell and the loss of important organic molecules from the cell result in irreversible cell damage. The efficacy of some of the drugs can be related to their ability to bind to ergosterol. However, the polyenes also are associated with the formation of lethal reactive oxygen molecules. Polyenes also are defined according to the concentration of drug necessary to cause erythrocyte hemolysis, presumably because of cholesterol binding. Pimaricin causes lysis at low concentrations, limiting use to topical application, whereas amphotericin B and nystatin cause lysis at much higher concentrations.3
Amphotericin is fungistatic but can be fungicidal at high concentrations. At high concentrations the drug directly disrupts the fungal cell membrane. As with some other select antifungal drugs, amphotericin appears to have some immunomodulating characteristics. Both humoral and cell-mediated immunity may be enhanced, thus increasing the host’s ability to overcome infection. Amphotericin B activates macrophages and stimulates TNF-α and interleukin-1 (IL-1), both of which facilitate macrophage killing by way of nitric oxide–dependent pathways.5,14
Spectrum of Activity and Pharmacodynamics
Despite the advent of the azole antifungal drugs, amphotericin B remains the most effective agent against most of the major fungal pathogens. The indications for amphotericin therapy include most systemic fungal diseases, including those caused by the dimorphic fungi (histoplasmosis, blastomycosis, cryptococcosis, and coccidioidomycosis) and disseminated sporotrichosis, phycomycosis, aspergillosis, and candidiasis. Amphotericin B has greater activity against some organisms (e.g., Candida and Aspergillus species and coccidioidal meningitis) than the newer azoles and particularly fluconazole. Amphotericin B is not effective against dermatophytes. Amphotericin MICs appear to correlate well with efficacy in animal models and human patients.10 The MICs of amphotericin B toward selected fungal microbes are listed in Table 9-4.15,16 Although these are based on humans, they represent a reasonable target for isolates infecting animals as well. In addition to the MIC, the minimum fungicidal concentration (MFC) has been reported for selected organisms infecting humans (or animals).15 The MFC50 and MFC90; μg/mL) are, respectively, Blastomyces dermatitidis (0.125, 0.5); H. capsulatum (0.5, 2), and C. immitis (4, 16). In killing-curve studies, amphotericin B consistently exhibits concentration-dependent killing. However, killing is variable among organisms, being fungicidal toward Candida sp. but not toward many yeasts and other organisms. As a concentration-dependent drug, amphotericin B may exhibit a long post-antifungal effect (PAFE), which, like the postantibiotic effect of concentration antimicrobials, varies in duration with the organism and the relationship of the MIC to the plasma drug concentration (PDC).10 However, it can prolong the dosing interval of the drug. A PAFE of only 0.5 hours occurs at approximately 2 hours exposure at drug concentrations that reflect 0.5 times the MIC, but this duration is prolonged to approximately 10 hours at 32 times the MIC for Candida and Cryptococcus neoformans; the PAFE for the latter may exceed 12 hours.10
Sufficient data exist to integrate Ambisome pharmocokinetics and pharmacodynamics toward selected microbes that infect the dog (Table 9-4; see also Table 9-2). Two targets are considered: the MFC and a 12-hour PAFE (targeting PDCs that equal the MIC90 of the infecting microbe by 32). Based on the Cmax of dogs for Ambisome measured at day 1 and day 30 of dosing, the MFC of B. dermatitidis and H. capsulatum will be achieved on the first day of dosing at 1 mg/kg. Assuming the PAFE as described in animal models would be exhibited by dimorphic fungal organisms infecting dogs, based on MIC90 for B. dermatitidis of 0.05 μg/mL (see Table 9-2), a target of 1.6 μg/mL (32 times the MIC) is indicated to achieve a 10 to 12-hour PAFE. Again, the target will be reached in dogs on day 1 with a 1 mg/kg dose of Ambisome; the 12-hour PAFE could be added to the time the PDCs are above the MIC (which in turn, is based on half-life). For H. capsulatum, the MIC90 is 1 μg/mL; accordingly, a target of 32 μg/mL (for at least 2 hours) might be indicated to achieve a 10 to 12-hour PAFE. Assuming linear kinetics, reaching the target on day 1 will require a dose of approximately 4 mg/kg, which is likely not to be tolerated (an exception may occur for lipid-based products). However, the target is likely to be approached with 30 days of dosing at 2.5 mg/kg. C. immitis is less susceptible to the killing effects of amphotericin B; the MFC90 (11 μg/mL) is likely to be reached on day 1 of dosing at 2.5 mg/kg, but 30 days of dosing will be required to reach the MFC90 or the 12-hour PAFE. These calculations were based on the MIC90 of the respective organisms; if the MIC of the infecting microbe is known, then a lower dose might be reasonable.
Resistance
The incidence of resistance to amphotericin B is low and has been documented primarily for Candida. The development of resistance may be related to changes in the number of sterol components in the fungal cell wall, with low ergosterol content associated with resistance.3 Pythium insidiosum, classified as a pseudofungus, (the cause of what was previously and inappropriate referred to as fungal phycomycosis), as with other oomycetes, is largely devoid of cell membrane sterol. As such, they are inherently resistant to polyenes.1 However, other mechanisms of resistance also exist, probably reflecting multiple mechanisms. Some organisms are able to resist concentrations that exceed 2 μg/mL. The azole derivatives may contribute to amphotericin B resistance by preventing the formation of ergosterol, the target of amphotericin B.1
Preparations
Because amphotericin is unstable, it is prepared with desoxycholate as a lyophilized cake form (amphotericin B; previously Fungizone®; AmBD). Supplies were limited at the time of publication,8 perhaps reflecting declining use in human medicine in favor of newer lipid-based formulations.
Desoxycholate—also referred to as deoxycholate, a bile salt—is added to aid solubilization of the lyophilized cake form of the drug, which, when reconstituted, is in a colloidal suspension. Reconstitution should be with sterile water only; the solution will remain stable for 1 week if refrigerated. Storage should include protection from light, although protection during therapy is probably not necessary; potency is unaffected after light exposure of up to 24 hours. Labeled directions indicate that dilution should occur only with 5% dextrose to prevent drug precipitation and inactivation; Trissel’s Handbook on Injectable Drugs (http://online.statref.com/titles/titleinfopage.aspx?titleid=141) indicates that normal saline is incompatible with amphotericin B, leading to a 43% drop in drug concentrations within 2 hours. Although the drug has been mixed by veterinarians with 0.45% NaCl and 2.5% dextrose with no apparent change, the basis for this observation is unclear, and the prudent clinician would avoid the combination and provide sodium loading (see the section on therapeutic use) through a different catheter. In general, amphotericin B should not be mixed with other drugs; although known to be compatible with some, it is incompatible with many others. The drug should be administered only intravenously, with exceptions for localized treatment in selected body tissues or fluids (e.g., aqueous humor, cerebrospinal fluid [CSF]). For small animals, which do not require a complete vial, the reconstituted drug can be divided into smaller aliquots and frozen.
Amphotericin also has been complexed to lipid mixtures in an attempt to reduce nephrotoxicity. Reticuloendothelial cells phagocytize the lipid component, facilitating directed delivery to the site of fungal infection. Drug uptake by the hepatic and splenic macrophages, bone marrow, and inflammatory tissues is thus enhanced. For liposomal products, some studies have shown that amphotericin B is selectively transferred from liposomes to fungal but not host cell membranes. Prolonged antifungal activity (compared with nonliposomal preparations) has been documented for these preparations. Each product is injectable and differs in the lipid makeup as well as the ratio of lipid to amphotericin B. Lipid-based products include a lipid complex (ABLC; Abelcet) suspension that forms a tightly packed ribbonlike structure and is the largest of the lipid-based products (250 nm); a colloidal dispersion of cholesterol sulfate, which forms 122 nm disks (Amphotec or Amphocil [ABCD]); and a single-layered liposomal product, Ambisome (LAmB; see Table 9-4), which is the simplest and smallest (60 to 70 nm) of the molecules.17,18 The differences among these products have recently been reviewed.19 Amphotericin B also has been administered with a lipid vehicle (intralipid; AMB-IL) rather than 5% dextrose. Although nephrotoxocity appears to be reduced in some patients, it does not appear to be decreased in the patients at most risk for toxicity, and therefore liposomal lipid-based products should be considered.18a Administration of AMB-IL also is not recommended because of mixed product is unstable. The differences among the lipid-based or lipid-containing products and their clinical use in humans have recently been reviewed.19
Pharmacokinetics
Amphotericin is not water soluble and thus is not bioavailable after oral administration. It is more than 90% bound to circulating serum lipoproteins (including cholesterol). Penetration into the pleura, peritoneum, inflamed tissues, CSF, and aqueous humor may result in a drug concentration two thirds of that in the plasma. The metabolism and excretion of amphotericin is not well characterized and is complex, being complicated by binding to cholesterol, which is structurally similar to ergosterol (see Figure 9-3). Biliary elimination may be the primary method of excretion (in humans only 3% of the drug is eliminated in the urine); however, concentrations are detectable in both bile and urine for up to 7 weeks in human patients.
The pharmacokinetics of the various amphotericin B preparations, including those complexed to lipids, vary markedly in humans; interpretation of kinetics is complicated by the lack of discrimination between free amphotericin B and that complexed to the various carriers. Because of its small size, Ambisome is characterized by the slowest uptake, and the highest PDCs. In humans, peak PDCs (μg/mL) vary with the dose (indicated in mg/kg), reflecting differences in volume of distribution that vary on account of tissue uptake: AmBD, 2.9 μg/mL at 1 mg/kg); Albecet (ABLC), 1.7 μg/mL at 5 mg/kg); Amphotec (ABCD), 3.1 μg/mL at 5 mg/kg; and Ambisome (LAmB), 83 μg/mL at 5 mg/kg. For LAmB, concentrations appear to be both dose and time dependent in dogs (Table 9-4). An advantage of the liposomal product is that it accumulates at sites of infection and targets the fungal cell wall, with release of amphotericin into the cell.19 For ABLC (and LAmB), tissue or fungal phospholipases appear to release amphotericin B at the site of infection. However, the colloidal form of amphotericin B (Amphocil), which is taken up by reticuloendothelial cells, was demonstrated in humans to accumulate in lung tissues to a greater concentration than Ambisome, suggesting that it might be preferable for respiratory infections.20 This finding requires further support by animal model or clinical studies. That LAmB may be preferred for central nervous system (CNS) infections is supported by the study and review of Ibrahim and coworkers,21 who address the potential inability of ABLC to penetrate the brain in mice or rabbit models. Comparing dosing ranges, LAmB was effective without nephrotoxicity at 5 to 20 mg/kg in animal models of disease, with highest tolerated doses being 30 to 50 mg/kg (as reviewed by Adler).19 Although ABLC was efficacious at doses ranging from 5 to 15 mg/kg, nephrotoxicity and decreased efficacy occurred at 15 mg/kg compared to 5 mg/kg. (as reviewed by Adler).19
The pharmacokinetics of Ambisone (LAmB) have been described in Beagles (n = 10 per group) as part of a toxicity study in humans and compared historically to AmBD (see Table 9-4).22 Dogs received 0.25 to 16 mg/kg/day as an intravenous infusion over 5 minutes, with PDCs determined on days 1, 14, and 30. Dogs receiving 8 and 16 mg/kg daily did not finish the treatment period because of severe weight loss (n = 17/20); this same pattern of weight loss was also described in dogs receiving AmBD at 0.75 mg/kg every other day (as reviewed by Bekersky and coworkers22). However, dogs tolerated 4 mg/kg Ambisone daily with peak concentrations at this dose were markedly variable, ranging from 18 µg/mL at day 1 to 94 μg/mL at day 30. Concentrations of Ambisone were a hundredfold higher than other formulations: A dose of 0.6 to 0.75 of AmBD resulted in peak amphotericin concentrations of 1.25 to 4.4 μg/mL after multiple dosing. This compares to a peak concentration of 54 ± 16 μg/mL at 4 mg/kg daily. Ambisome kinetics were nonlinear, with clearance and distribution decreasing as dose increased, potentially reflecting saturation of distribution and clearance processes. For example, although dose only increased 64-fold in the study, area under the curve (AUC) on day 1 at 0.25 mg/kg was 2.6 μg∗hr/mL (compared) to 2592 μg hr/mL at 16 mg/kg, representing a 2500-fold increase. Multiple dosing also resulted in accumulation at each dose, with 30-day to 1 day AUC ratios being 2.5 and 10 at low (0.25 mg/kg) and intermediate (4 and 8 mg/kg) doses, respectively. (see Table 9-4). Kinetics are likely to be equally complex in other dogs and cats, and lack of predictability may limit use.
Toxicities and Side Effects
Nephrotoxicity is the major side effect associated with the use of amphotericin B. Renal function becomes impaired in more than 80% of patients receiving amphotericin,4,23,24 with serum creatinine at least doubling in 40% to 60% in human.23 Although largely reversible, renal toxicity depends on total cumulative dose and duration of therapy. In human patients chronic renal disease occurs in close to 50% of patients receiving more than approximately 60 mg/kg total dose compared with only 17% who receive less than 57 mg/kg. The proportion decreases to 8% in those receiving less than 14 mg/kg total dose.23 Although renal function usually returns to normal before completion of therapy, some residual damage often persists after discontinuation of the drug. Two mechanisms are important in renal toxicity. Intense arterial vasoconstriction occurs within 15 minutes of administration and lasts 4 to 6 hours. The mechanism is unknown but can lead to acute tubular nephrosis secondary to ischemia. Nerve degeneration, angiotensin II receptor blockage, adrenergic blockade, and potent vasodilators do not prevent renal vasoconstrictive effects. Amphotericin B may activate formation of vasoconstrictive (thromboxane) arachidonic acid metabolites, which suggests that direct vasoconstriction may be responsible. Amphotericin B can increase calcium fluxes. Histologic lesions are most profound in regions vulnerable to hypoxia or areas rich in oxygen.23
Distal renal tubular toxic effects result from binding of membrane cholesterol in the tubular cell membrane (see Figure 9-4). Altered electrolyte fluxes result in acidification abnormalities (metabolic acidosis), hypokalemia, and concentrating defects (polyuria, polydipsia). Renal tubular acidosis is common, dose related, and generally reversible (although resolution may take several months) and generally precedes significant decreases in glomerular filtration rate (GFR). Potassium and magnesium wasting in humans can lead to substantial deficits.23 Magnesium deficits appear at 2 weeks and are maximal at 4 weeks. Both potassium and magnesium should be monitored; electrolyte abnormalities may persist for several weeks after therapy is discontinued. Rarely, hyperkalemia has been reported in association with rapid infusion as potassium shifts from the intracellular compartments. The risk is greater in patients with renal failure. Concentration defects occur in essentially all human patients, are not related to azotemia, and may persist for months. Urine specific gravity may not be an effective measure by which to assess renal damage.
Ampohtericin B toxicity has been documented in dogs. Ceylan and colleagues24 described the nephrotoxicity of AmBD in dogs (n = 18; cross-bred) receiving 0.5 mg/kg in 25 mL 5% dextrose in water as a 4- to 5-minute intravenous bolus; 1 mg/kg in 50 mL as a 4- to 5-minute bolus, or 2 mg/kg in 1000 mL over 4 to 5 hours. Dogs were treated for 12 days. Side effects (vomiting, diarrhea, anorexia, fever, tachycardia, and phlebitis) were evident in all dogs by day 3 but were worse in dogs receiving 1 mg/kg and least in dogs receiving 2 mg/kg. Indicators of renal damage (blood urea nitrogen, creatinine) were increased in all groups by day 5 but were highest in the 1 mg/kg group. Serum calcium was significantly decreased compared with baseline in the 1 mg/kg and 2 mg/kg groups. Hematologic indicators of anemia were evident in the 1 mg/kg group. Among the indicators of nephrotoxicity studied was urine γ-GGT, which was significantly higher than baseline in all groups by day 5 of therapy but did not differ among groups or times during treatment. On the basis of this study, the authors suggested that infusion over a 4- to 5-hour period (or fluid support) decreases the risk of aminoglycoside toxicity. However, no study has determined the long-term effects of any method of administration of amphotericin B on renal failure in dogs or cats.
In their pharmacokinetic study of LAmB (Ambisome) in Beagles at doses ranging from 0.25 to 16 mg/kg daily for 30 days, Bekersky and coworkers22 reported that no dogs died as a result of the drug; however, dose-dependent tubular nephrosis was present at 1 mg/kg, and 70% and 100% of dogs in the 8 and 16 mg/kg treatment group, respectively, were euthanized before the end of the study because of 25% or more body weight loss. Vomiting and diarrhea were evident in all groups, including a liposomal (i.e., without amphotericin B) control, but were worse in the 4, 8, and 16 mg/kg treatment groups. Azotemia was not present in the 1 mg/kg group. Azotemia was described as moderate and clinically significant toward the end of the 30-day treatment period in the 4 mg/kg group, with creatinine increasing from a baseline of 0.7.1 ± to 2.3 ± 0.4 mg/dl by study end. Serum potassium was not clinically affected. The authors concluded that dogs tolerated up to 4 mg/kg well, despite peak amphotericin B concentrations ranging from 18 to 94 μg/mL.
Acute anaphylactic-type reactions such as vomiting, fever, and chills can occur with the use of amphotericin B and have been reported with lipid complex preparations as well.25 Up to 30% of dogs receiving AmBD develop fever.26 The frequent incidence of these reactions often leads to pretreatment for anaphylaxis (one-time use of a short-acting glucocorticoid does not enhance the toxicity of amphotericin B; see the discussion of “cocktail” in the following section).
Drug Interactions
In general, drugs that are renally active are not recommended for patients also receiving amphotericin B. In humans cyclosporine and tacrolimus (calcineurin inhibitors) will enhance nephrotoxicity. However, these immunomodulators tend to be safer, as far as nephrotoxicity is concerned, in both dogs and cats, and the combination may not be as dangerous. The nephrotoxicity of amphotericin also may be enhanced with catabolic drugs such as glucocorticosteroids, antineoplastic drugs, antiprostaglandins (glucocorticoids and nonsteroidal antiinflammatory drugs), and other nephrotoxic antibiotics. One study demonstrated that diuretic therapy during, but not just before, amphotericin B therapy increased the risk of nephrotoxicity more than twelvefold (as reviewed by Bagnis and Deray23). Although diuretics have been used for a “protective” effect, the potential benefits are limited to mannitol, and even it may be associated with electrolyte disturbances. Combination with digoxin may enhance nephrotoxicity and hypokalemia. Declining renal function in association with amphotericin B therapy may affect clearance of co-administered drugs. Because it is renally excreted, 5-flucytosine toxicity may be enhanced if renal function declines with amphotericin B.
Therapeutic Use
Multiple strategies may help reduce toxicities or side effects to amphotericin B. The first focuses on prevention of an anaphylactoid reaction by pretreatment (to prevent vomiting, fever, chills, and anaphylaxis) with antihistamines (diphenhydramine 0.5 mg/kg, administered intravenously) and short-acting glucocorticosteroids (e.g., hydrocortisone sodium succinate, 0.5 mg/kg, administered intravenously). Because the reaction appears to be associated with direct mast cell degranulation (because of the cationic nature of amphotericin B), pretreatment with a small test dose (0.1 mg/kg for cats or 0.25 mg/kg for dogs diluted in 10 mL infused over 15 minutes) may help identify animals that are likely to have an adverse reaction during infusion. Whereas lipid products may be characterized by less nephrotoxicity, they are more likely than nonlipid products to cause vomiting, nausea, and phlebitis.38 As such, pretesting is indicated with their use as well.
In addition to dilution protocols (see discussion under Toxicity), strategies are intended to minimize amphotericin-B induced nephrotoxicity. Although what constitutes amphotericin B nephrotoxicity may vary, an increase in creatinine of as little as 25% may be considered significant in some patients.18 Azotemia precedes tubular dysfunction in humans, allowing for early detection (i.e., before irreversible damage). Urine sediment initially should be monitored for evidence of nephrotoxicity. Serum chemistries tend to be less sensitive indicators of nephrotoxicity. Urine γ-gt has been used to assess lipid-based amphotericin B toxicity. The drug should be temporarily discontinued (24 to 48 hours) if the blood urea nitrogen level becomes abnormal.27
First and foremost, pretreatment with sodium-containing fluids is particularly important for preventing renal toxicity, including that associated with renal arterial vasoconstriction; posttherapy treatment might also be prudent. Secondly, amphotericin B can be administered with a “cocktail” intended to protect the kidney. Administration of the dose diluted in 5% dextrose is accompanied by mannitol (0.5 mg/kg) to maintain glomerular filtration rate and sodium bicarbonate (1 to 2 mg/kg) to prevent cellular acidification defects. The mannitol and sodium bicarbonate should not be added directly to the amphotericin B solution but given through another catheter in order to avoid precipitation of amphotericin B. The ability of cocktails to prevent nephrotoxicity is controversial. The treatment is based on one small study in dogs31 for which mannitol was demonstrated to protect the kidney. However, a subsequent study (using isolated perfused rat kidneys) found mannitol to impart a protective effect, presumably by reducing renal tubular edema by virtues of its osmotic effect.32 Binding of cellular cholesterol prevented toxicity, supporting the presumed mechanism of direct cytotoxicty. This study also demonstrated a synergistic toxic effect of amphotericin B in the presence of hypoxia. Only one clinical trial, in humans, has addressed renoprotection by mannitol, and it failed to identify an effect. However, this clinical trial, although randomized, involved only 11 patients and is probably not conclusive (as reviewed by Bagnis and Deray18,23) Although such cocktails generally are not harmful as long as the solutions are not mixed with amphotericin B, mannitol has been associated with electrolyte abnormalities in the human-medicine literature.23 Deray18 indicates that mannitol decreases renal medullary blood flow and PO2. As such, the use of mannitol remains controversial. A relatively recent study describes the nephroprotective effects of N-acetylcysteine (10 mg/kg daily) in a rat model of amphotericin-induced nephrotoxicity;33 administration began 1 day before amphotericin was started. As previously described, amphotericin should not be mixed with solutions containing electrolytes, acidic solutions, or preservatives because these materials may cause drug precipitation. The rate at which amphotericin B is administered may also reduce the incidence of nephrotoxicity.
The third strategy by which amphotericin B toxicity might be reduced is administration with lipids or as a specialized delivery system (in lipids). Conventional amphotericin B is not recommended in humans in the presence of renal insufficiency, hypokalemia, hypomagnesemia, tubular acidoses, or polyuria.18 Lipid preparations are designed to deliver more drug selectively to the site of infection,34 thus allowing higher doses (see the section on preparations). The liposomal products tend to be very expensive. In contrast to liposomes, fat emulsions are easy to prepare and administer and tend to be more cost effective. These products appear to be equal in efficacy to nonliposomal products but safer with regard to nephrotoxicity. However, the use of intralipid as the vehicle (AmB-IL) is associated with other difficulties that limit its use (see previous discussion).
The safety of liposomal or lipid-based products may allow delivery of higher doses without an increased risk of nephrotoxicity, with the incidence of nephrotoxicity reduced by 8% to 28%, depending on the preparation.18,23 Although several studies are available that address the safety of these products individually, and LAmB (Ambisome) in particular,35 none appears to have compared all three products. However, in general, the liposomal product LAmB (Ambisome) is recognized to be the safest with regard to nephrotoxicity. Ambisome achieves the highest concentrations in humans compared with other amphotericin preparations but is well tolerated renally.17,19 In general, efficacy of LAmB is equal to or exceeds that of AmBD and is consistently better tolerated. Survival rates in humans for the product ranges from 99% to 100% (visceral leishmaniasis; three studies, n ≅ 400), 37% to 78% (aspergillosis; five studies, n ≅ 400), 84% to 93% for cryptococcosis (two studies, n ≅ 280), 98% for histoplasmosis (one study, n = 55), and 20% to 69% for zygomycosis (three studies, n ≅ 130).35 That LAmB may be preferred for CNS infections is supported by the study and review of Ibrahim and coworkers,21 who found LAmB generally superior to ABLC in treating CNS infections, as indicated by response and decrease in fungal load. Abelcet (ABLC) and LamB were generally characterized as having the lowest incidence of all side effects (fever, chills, nausea, dyspnea, hypertension, hypotension, tachycardia, and nephrotoxicity) with the exception of hypokalemia (42% with LamB). Ambisome was associated with abnormal hepatic function tests in approximately 20% of human patients.17 Abelcet causes fewer infusion-related side effects in humans, and therefore a test dose is not necessarily indicated, whereas Amphotec is more likely to cause infusion side effects and should be given as a short infusion only.
One study reports the efficacy and safety of a liposomal product when used at higher than recommended cumulative doses for treatment of canine blastomycosis.36 In another study, although the products were equally efficacious, the degree of nephrotoxicity between a fat emulsion and standard amphotericin B was no different.37 Amphotericin B also has been administered with a lipid vehicle (intralipid) rather than 5% dextrose. Although nephrotoxocity appears to be reduced in some patients, it does not appear to be decreased in the patients at most risk for toxicity, and therefore liposomal lipid-based products should be considered.18 Further studies documenting the efficacy and safety of liposomal or fat emulsion products containing amphotericin B are needed.
A fourth strategy that can be used to minimize adverse events associated with amphotericin B is administration using alternative routes. Localized mycotic infections have been treated with localized administration of amphotericin, which may reduce the incidence of nephrotoxicity. Subconjunctival, intravitreal, intrathecal, intranasal (human aspergillosis: 5 mg/mL in water administered as aerosol), and intraperitoneal routes have been reported. Oral administration has been used for treatment of gastrointestinal candidiasis and presumably might be used for other gastrointestinal fungal disorders.29 Both LamB and ABLC have been demonstrated to have enhanced efficacy when administered as an aerosol for pulmonary infections (as reviewed by Adler).19 Amphotericin B (AmBD) can be mixed in sterile water to 200 mg/kg and infused into the bladder for fungal cystitis. For fungal infections of the CNS, the drug can be given (0.2 to 0.5 mg in either 5 mL of CSF or 10% dextrose) intrathecally (under general anesthesia) two to three times per week.27
Combination antifungal therapy is a fifth stategy that is strongly encouraged to enhance efficacy and thus decrease the duration of antifungal exposure to the host. For example, as reviewed by Adler,19 efficacy of LamB is enhanced when combined with micafungin (zygomycosis, aspergillosis) and voriconazole (aspergillosis).
Although a sixth strategy whereby amphotericin B toxicity can reduced includes variation in doses, frequency of administration, concomitant therapy, and duration of therapy, no protocol has proven to be superior to others. Some literature supports rapid intravenous administration (bolus) in less debilitated dogs; slow intravenous administration might be more prudent in cats. A dose (0.25 to 0.5 mg/kg) can be diluted in 300 to 1000 mL of 5% dextrose and administered in an indwelling catheter over 2 to 6 hours, or it can be diluted in as little as 10 to 60 mL (30 recommended) and given over 2 to 10 minutes through a butterfly catheter27 (flush catheter after infusion). The slow infusion method has the added advantage of additional fluids, which may reduce the incidence of nephrotoxicity,28 especially in debilitated animals. The advantages of dilution and slow infusion is preferred and was previously discussed (see Toxicity). It is recommended that the small bolus be preceded or followed up with supplemental fluid, preferably normal saline. One report of an uncontrolled clinical trial describes the successful and apparently safer administration of amphotericin B after twice- or thrice-weekly subcutaneous administration (0.5 to 0.8 mg/kg diluted in 400 to 500 mL of fluid such that amphotericin B is less than 20 mg/L) for several months for treatment of cryptococcosis in dogs and cats.30 However, only five successful cases were described, and further confirmation should be expected before this route is routinely embraced.
The sequence of repetitive treatments is also controversial. Some authors recommend alternate-day therapy, whereas others recommend daily therapy at a smaller dose. Doses also vary. Daily doses range from 0.15 to 0.5 mg/kg every other day (e.g., on Monday, Wednesday, Friday) until a cumulative dose of 4 to 12 mg/kg (depending on the organism or if therapy is combined with another antifungal) AmBD has been reached. Starting at a low dose (0.15 to 0.25 mg/kg) and gradually increasing the dose until the desired daily dose has been reached may reduce the severity of side effects. For particularly resistant infections, a dose of 1 mg/kg has been used on an alternate-day basis.
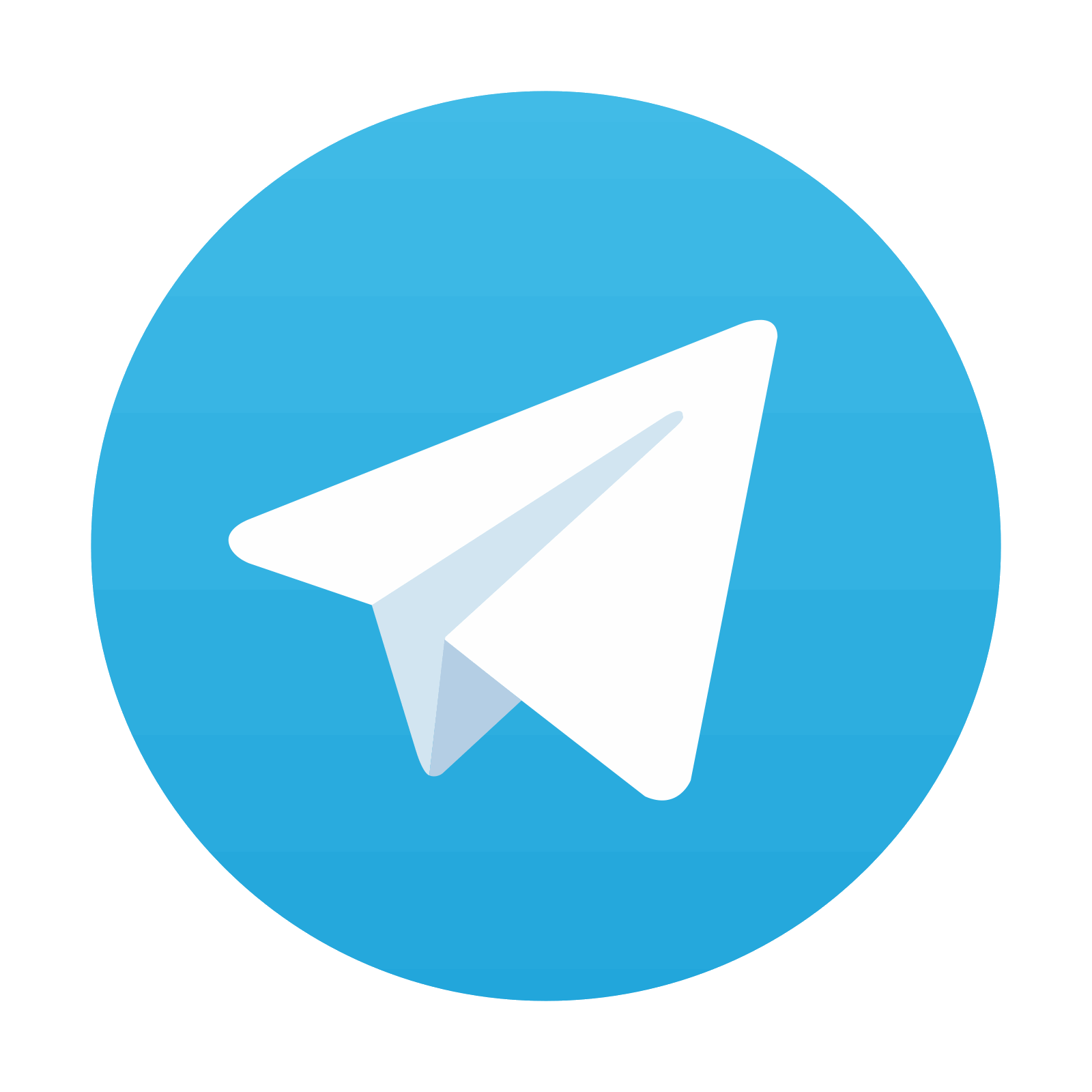
Stay updated, free articles. Join our Telegram channel
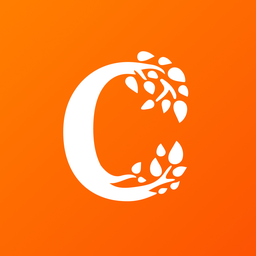
Full access? Get Clinical Tree
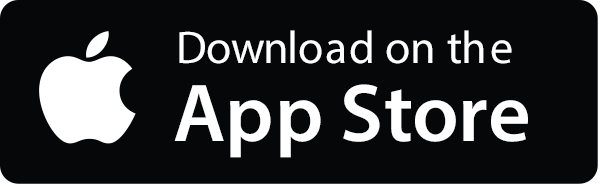
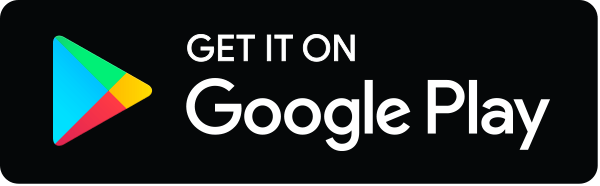