8 Treatment of Bacterial Infections
This chapter focuses on the treatment of bacterial infections on a systems basis. In addition, selected organisms are discussed because of their unique nature and the difficulties encountered when treating such infections. In general, the ease with which microbes appear to become resistant requires dosing regimens that are based on scientific studies demonstrating both efficacy toward the microbe and pharmacokinetics in the target species; if the latter is not available, extrapolated doses should be promulgated by persons with expertise (e.g., a veterinary clinical pharmacologist). Because of limited evidence-based information in veterinary medicine, data from the human-medicine literature may serve as a basis for recommendations. Information supporting the judicious use of antimicrobial drugs is found in Chapters 6 and 7. This includes but is not limited to data referring to drug concentrations achieved in the plasma at recommended doses (see Table 7-7), and other pharmacokinetic data (see Table 7-1) and tissue-to-drug ratios of antimicrobial drugs (see Table 7-5). In addition, population pharmacodynamic data are available that indicate the concentration of drug necessary to inhibit the microbes (minimum inhibitory concentration [MIC]) for selected drugs: See Table 7-3 (Escherichia coli), Table 7-4 (human data for selected microbes for which veterinary information could not be consistently found), Table 7-9Table 7-10 (data for beta-lactams), and Table 7-12 (fluoroquinolones). Selected pharmodynamic data are also provided in this chapter for infections associated with specific body systems. This includes an example of regional cumulative antimicrobial susceptibility antibiograms that might help guide empirical therapy, which is increasingly discouraged as resistance emerges (Figure 8-1). The clinician is encouraged to consider the approach to individualized antimicrobial therapy presented in Chapter 6. This includes Figure 6-1, which is an algorithm for antimicrobial therapy that is intended to minimize the risk of antimicrobial resistance, particularly in the at-risk patient. Algorithms have also been offered for infections of selected body systems. Preventing resistance is among the more important considerations that must be made by veterinarians using antimicrobials. (Box 8-1). A three “Ds” approach includes “detergent” (address hygiene at multiple levels), “de-escalating” drug use, and “design” dosing optimal regimens. Hygiene, which goes beyond simple hand washing, also is a critical component of preventing emerging resistance. Resistance is also minimized by de-escalating drug use. This includes simply decreasing the number of antimicrobial drugs prescribed and dispensed. However, de-escalating also entails moving to a lower tier of drug classes (i.e., one that is less broad in its actions and less “effective” toward microbes that tend to develop multidrug resistance). Although it is critical to use the best drug possible such that the infecting population is killed, once this is accomplished, de-escalation to a less-important lower tier class of drugs should be possible. First-tier drugs might include both narrow and broader spectrum beta-lactams (amoxicillin with without clavulanic acid), clindamycin, tetracyclines, and potentiated sulfonamides. Second-tier drugs might include those drugs characterized by spectra purposefully extended to target organisms not generally susceptible to first-tier drugs. Newer drugs such as third-generation cephalosporins, extended-spectrum penicillins, and fluoroquinolones might be included in this category. Therapy should be based on culture and susceptibility testing data, whenever possible, and population pharmacodynamic statistics if patient MIC data are not available. Third-tier drugs include those that tend to be reserved for treatment of microbes associated with either inherent or acquired resistance. Their use should be based on culture and susceptibility testing data and should be de-escalated to a lower-tier drug as soon as possible. Examples might include drugs that target multidrug-resistant gram-negative (aminoglycosides, carbapenems) and gram-positive (glycopeptides, linezolids) organisms. The American College of Veterinary Internal Medicine1 and the International Society of Companion Animal Infectious Disease (publications pending) have promulgated guidelines intended to minimize emerging resistance.
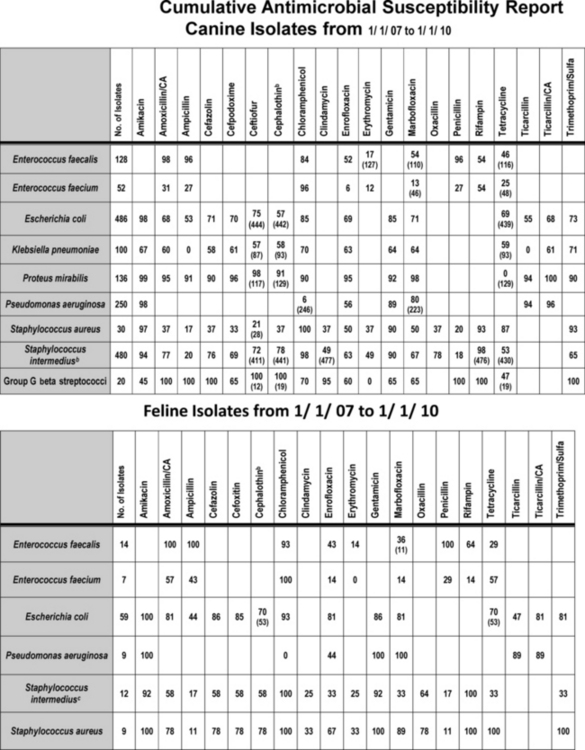
Figure 8-1 Cumulative antimicrobial susceptibility reports for canine (top) and feline (bottom) organisms isolated from samples collected during the years 2007 to 2010 at a veterinary teaching hospital. The data are geographically restricted to Alabama and are from a teaching hospital that has a wide referral base. Accordingly, the data may not be relevant to other areas of the United States nor to patients with first time infections. No attempt has been made to separate data according to history, including previous antimicrobial exposure. Ideally, each practice would generate a cumulative report at frequent intervals (e.g., yearly or every other year). The report might serve as a basis for empirical selection of antimicrobials. However, the number of isolates must be sufficient to represent the population; ideally, at least 100 isolates of each organism should be sampled. Drugs that are not included in the antibiogram for each organism generally are not included because the use of the drug for that organism is inappropriate. Each cell indicates the percentage of the tested isolates that were considered susceptible to the drug on the basis of Clinical and Laboratory Standards Institute (CLSI) guidelines promulgated in 2008. The first row lists the drugs to which susceptibility was determined for the organisms. The far left column names the genus and species of the organisms isolated and for which susceptibility was determined. The second from the left column indicates the total number of isolates tested. For some drugs not all isolates were tested; in such instances the total number of isolates tested is indicated in parentheses in that cell. New CLSI interpretive criteria for selected drugs (approved in 2010), including amoxicillin–clavulanic acid cephalexin and its model drug, cephalothin, are likely to result in a marked decrease in the percentage of susceptible isolates. Cephalothin continues to act as a class drug, representing first-generation cephalosporins (see Chapter 6 for a discussion of limitations of model drugs; e.g., cephalexin should not be used to treat E. coli, as a general rule). Staphylococcus intermedius represents what is currently referred to as S. intermedius group.
(Data provided by Terri Hathcock, MS, Diagnostic Veterinary Microbiologist, Infection Control Officer, College of Veterinary Medicine, Auburn University.)
Box 8-1 Treatment of Multidrug-Resistant Microorganisms
Infections of the Central Nervous System and Special Senses
Meningitis
Meningitis serves here as the prototypic infection of the central nervous system (CNS).
Physiology and Pathophysiology
The course of CNS infection is affected by the relationship of the brain and spinal cord to the vasculature, meninges, and skeletal structure. The brain is suspended in cerebrospinal fluid (CSF) and is surrounded by the meninges (pia mater and arachnoid [together forming the leptomeninges] and the dura mater). Infections of the leptomeninges tend to involve their entire surface that surrounds the brain and spinal cord. In contrast, infections of the dura mater tend to be limited and sharply circumscribed. With persistent infection of the meninges, increased intracranial pressure results from extensive cerebral edema and hydrocephalus. Infections of the spinal cord meninges are less limited and often extend longitudinally the length of the cord.1
About 85% of CSF is produced by the choroid plexus of the lateral, third, and fourth ventricles. The CSF flows into the subarachnoid space, circulates around the brain and spinal cord by bulk flow, and is reabsorbed through the arachnoid. The CSF is totally recirculated in 3 to 4 hours. The choroid plexus is physiologically similar to renal tubules, even containing similar secretory mechanisms. Indeed, specialized transport systems allow the movement of organic acids (including many beta-lactams) against a concentration gradient out of the CSF. In cases of infections involving the ventricles, because of the flow pattern of CSF, intrathecal administration of drugs does not result in predictable drug concentrations in the ventricles. Rather, drug must be directly instilled into the ventricles. Infections of the CNS can impair CSF reabsorption across the arachnoid villi, resulting in hydrocephalus.1
Capillaries of the brain and spinal cord (with the exception of the choroid plexus) differ from other capillaries. First, the vascular endothelium is characterized by tight junctions rather than intracellular clefts. Second, they are surrounded by the foot processes of astrocytes (see Figure 29-1). Both form a barrier to passive diffusion of drugs and their compounds. Only compounds that are actively transported or are of sufficient lipid solubility can pass out of the capillaries into the brain. The barrier affects the movement of antimicrobials. In addition, impaired movement of immunoglobulins, complement, and other mediators of the immune response affects antimicrobial selection in that bacteriostatic drugs are much less desirable.1,2
Vascular damage associated with infection can affect the course of infection. Hypertrophy of the endothelium (as might occur with persistent bacterial infection) or infection of the endothelial cells (as occurs with Rocky Mountain spotted fever, for example) can cause thrombosis or embolization to arteries or veins. Loss of capillary integrity contributes to cerebral edema and movement of microorganisms into the brain. At the same time, capillary permeability facilitates movement of antimicrobials that normally cannot cross the cerebral or meningeal capillaries into the site of infection.1,2
The inflammatory response of the CNS also differs from that in other body tissues. The response tends to be less intense and is characterized by infiltration of microglial cells and proliferation of astrocytes. Abscessation is slower and involves gliosis rather than fibrosis. Host response to infection in the CNS involves antibody, cell-mediated immunity, and complement-mediated immunity. Normally excluded from the CNS, antibody in the CNS indicates damage to the blood–brain barrier or synthesis of immunoglobulin from cells that have been able to penetrate the brain parenchyma. Antibody protection is important in bacterial meningeal infections and may determine the outcome. Cell-mediated immunity, on the other hand, is the predominant host response to fungal or intracellular parasites. Infections by selected organisms, such as Mycoplasma spp., may lead to a host response to both the infecting organism and host proteins (e.g., myelin). Despite the role of the immune system in bacterial infections of the CNS, host defenses remain inadequate for control of the infection. Indeed, the relative lack of opsonization, complement, and immunoglobulins may allow bacterial survival in the subarachnoid space.1,2
Bacterial products can contribute to the development of cerebral edema. Release of cytokines and tumor necrosis factor is mediated by materials such as endotoxin of gram-negative organisms and teichoic acid produced by Staphylococcus aureus. Whereas changes in capillary permeability may increase antimicrobial movement across the blood–brain barrier, antimicrobial therapy may initially worsen cerebral edema, as bacterial death causes release of more mediators of inflammation. Inflammation, hemorrhage, hydrocephalus, and edema may cause displacement of the brain or spinal cord. Herniation may be a life-threatening sequela.1 The potential release of endotoxin may be an important consideration in the initial selection of an antimicrobial; drugs that minimize endotoxin release yet still penetrate the blood–brain barrier include meropenem and the fluoroquinolones.
Antimicrobial Selection
Successful antimicrobial therapy of CNS infections is facilitated by use of a bactericidal drug and maximization of plasma drug concentrations such that bactericidal concentrations are achieved in the CNS. The CNS is relatively immunoincompetent, thus increasing the concentration of drug necessary for effective therapy. Studies in animal models have shown that the rapid bactericidal killing in the CSF requires drug concentrations that exceed the minimum bactericidal concentration (not MIC) by tenfold to twentyfold.2 To maximize drug concentrations at the site of infection, drugs that can be given intravenously are preferred to oral preparations. Antimicrobials to treat the CNS are often selected empirically because of difficulties encountered when collecting culture and susceptibility data. Most infections reach the CNS by a hematogenous route. Organisms most likely cultured from or infecting the CNS are delineated in Table 6-1; however, the lack of predictability of the infecting organism mandates the need for a properly collected culture sample. After the most likely infecting organism and drugs effective against the organism have been selected, antibiotics should be selected next on the basis of movement into the CNS. Drug penetration of the blood–brain barrier is particularly challenging because the barrier not only prevents movement of antimicrobials into the CNS but also actively transports out or destroys some antimicrobials (i.e., selected beta-lactams) (see Table 7-5).
To enter the CNS, antimicrobials must penetrate the epithelium of either the choroid plexus or the cerebral endothelium; both are characterized by tight junctions. Antimicrobials generally are not metabolized in the CNS; concentrations thus reflect a balance between penetration and elimination via the blood–brain or blood–CSF barrier.3 Passive diffusion is the major mechanism of drug movement. Drugs that are more likely to penetrate the barrier are characterized by high lipid solubility, small molecular weight, and low protein binding.2 Whereas lipid-soluble drugs enter the CNS through transcellular pathways, water-soluble drugs must move through paracellular pathways and thus depend on the opening of tight junctions. Several transport mechanisms facilitate influx (as well as efflux; see below and Chapter 27) of selected drugs (e.g., penicillins, ceftriaxone), but this accounts for only a low concentration of drug movement. Antimicrobial movement into the CNS thus is generally slow, with peak concentrations often not occurring until several hours after drug administration. Methods intended to increase permeability of and thus antimicrobial movement into the CSF (e.g., hyperosmotic solutions, receptor-specific antibodies, inflammatory mediators) have not been well evaluated.3 Once selected drugs successfully penetrate the CNS, drug efflux may decrease intracellular concentrations. Penicillins (but not ceftriaxone, carbapenems, nor ampicillin) are actively transported from the CNS; active transport can be inhibited by probenicid.4 Interestingly, the action of this pump is inhibited by meningeal inflammation. Therefore inflammation will increase inward movement of several antimicrobial, including many beta-lactams and vancomycin. However, as treatment is effective, increased influx declines such that within 5 days of therapy, penicillin CSF half-life and drug concentrations in humans markedly decrease. This is in contrast to drugs with a low affinity for active transport system; for such drugs concentrations tend to remain clinically relevant throughout CNS infection. Active transport mechanisms do not appear to affect CSF concentrations of fluoroquinolones or aminoglycosides.3
Antimicrobial therapy also is likely to be affected by the presence of purulent material. As in all body systems, the microenvironment can negatively affect antibacterial therapy. In the presence of meningitis, lactate accumulates in the CSF, causing the pH to decrease. Antibacterial activity of weakly basic antimicrobials may decrease, particularly for the aminoglycosides and potentially for the fluoroquinolones.2 The dynamics of CSF are altered by disease and drugs. At best, CSF production is unaltered, although several studies have demonstrated decreased CSF production. Gluocorticoids may further decrease CSF production; however, rather than prolonging the half-life of the drug in the CNS, glucocorticoids appear to decrease or not change CSF antimicrobial concentrations.5
Drug movement into the CNS was summarized in Box 6-4 and Table 7-5. Because of the impact of normal physiology and drugs, dosing regimens designed for treatment of CNS infection tend to differ from traditional therapy, in that doses often are much higher. The risk of toxicity that might accompany such increases must be weighed against the need to penetrate the CNS in concentrations sufficient to cause bactericidal effects (see Table 7-5). In general, selected beta-lactams (meropenem more than imipenem; cefoxitin, ceftazidime and cefotaxim), trimethoprim/sulfonamide, fluorinated quinolones, rifampin, and metronidazole can achieve bactericidal concentrations for some infections in the CNS; chloramphenicol and doxycycline or minocycline achieve bacteriostatic concentrations.6 Amikacin may achieve effective concentrations as well. Drugs that should be avoided or whose doses should be further markedly increased for treatment of CNS infections because of poor penetration include many beta-lactams, including carbenicillin, cephalothin, cefazolin, cefotetan; and clindamycin, erythromycin, and tetracycline. Drugs recommended for treatment of meningitis in humans are increased by at least 50% to 100% or more when safety is not an issue, with intervals being reduced for time-dependent drugs, to ensure that adequate concentrations reach the CNS. For other drugs, doses for treatment of CNS infections are increased several-fold compared with other infections. For example, beta-lactam doses in particular are increased as follows: penicillin normally dosed at 1 million U is increased 1 to 4 million U to 24 million U/person; aztreonam is increased threefold, and several third-generation cephalosporins (cefotaxime, ceftazidime, ceftriaxone) are increased sevenfold to twentyfold. Aminoglycoside doses are either not increased or increased twofold (e.g., amikacin). Doxycycline is increased twofold, and sulfonamides are increased fourfold to fivefold. Because of local immunoincompetence, duration of therapy for patients with infections of the CNS should be at least 10 to 14 days and up to 21 days.2 The need for 4 to 6 weeks of therapy, as has been suggested in dogs, is not clear; it may not be necessary.7 Intrathecal administration of antimicrobials that might be systemically toxic at concentrations necessary to be effective in the CNS might be a reasonable alternative. However, this method has not been well studied, particularly in animal patients. Further, because distribution of intrathecally administered drug into the CSF is uneven, drug concentrations may not be adequate at some sites.
Adjuvant therapy
Because of the harm associated with inflammation in a closed system, antiinflammatories should be considered when treating CNS infections (e.g., meningitis). Corticosteroid therapy may be indicated during initial stages of treatment of meningitis to minimize the effects of inflammation and loss of capillary integrity.2,7 Experimentally, methylprednisolone decreases leukocyte accumulation, CSF outflow resistance, and brain water content in animals with bacterial meningitis.2 Dexamethasone also reverses the development of brain edema and, compared with methylprednisolone, has the added advantage of decreasing CSF pressure and lactate. Note that these studies did not include comparisons with antimicrobial therapy. Nonetheless, glucocorticoid therapy may be beneficial early during the course of therapy; indeed, treatment before antibacterial therapy may minimize the effects of mediators of inflammation released by dying bacteria.2 A study of infants with bacterial meningitis treated with ceftriaxone and either a placebo or dexamethasone found the duration of infection and degree of inflammation in the latter group to be shorter and less, respectively, although mortality or long-term neurologic sequelae did not differ between the two groups.8 In an analysis of five clinical trials in adult humans with bacterial meningitis, the incidence of side effects was the same in the group treated with glucocorticoids (dexamethasone), but both mortality and persistence of clinical signs were improved in the group treated with steroids, leading the authors to conclude that a single dose is justified if given at the beginning of antimicrobial therapy.9,10 Dexamethasone can be used (0.1 to 0.15 mg/kg every 6 hours up to 4 days), particularly in the presence of cerebral edema.2
Adverse reactions
Because of altered permeability of the blood–brain barrier, the infected CNS is more likely than the normal CNS to respond adversely to antimicrobials. Seizures are the most likely manifestation. The antimicrobial most likely to cause seizures include selected beta-lactams (see Chapters 6 and 7), most notably imipenem (but not meropenem), metronidazole, and fluoroquinolones, particularly in patients also receiving nonsteroidal antiinflammatories. In general, seizures that develop as a result of drug therapy should be treated as with any acute seizural manifestation, with diazepam the preferred anticonvulsant of choice.
Ocular Infections
The principles of ocular therapy are discussed in Chapter 27.
Microbial Targets
Whitley11 has provided a review of isolates cultured from ocular tissues of clinically normal dogs and dogs with ocular disease (Table 8-1). The most common organism associated with bacterial conjunctivitis in dogs is Staphylococcus spp. (aureus [68% of infections] or epidermidis [27% of infections]). A variety of other organisms, including Corynebacterium spp. and gram-negative rods, make up the remaining infections. Most of the infecting organisms are considered normal ocular flora.11 Bacterial infection complicating keratoconjunctivitis sicca generally is caused by Staphylococcus spp. (32% to 69% of dogs) and Streptococcus spp. (9% to 25%); Pseudomonas spp. (5% to 18% of infections) is the most common gram-negative isolate. In cats Mycoplasma felis and gatea are the more common isolates associated with conjunctivitis; however, they also are commonly isolated in normal cats, calling into question the role of the organism in disease. Chlamydia (Chlamydophila felis)12 may be a concurrent pathogen, although both it and Mycoplasma spp. may reflect infection secondary to primary feline herpesvirus infection.
Table 8-1 Microbial Flora Associated with Infections at Selected Tissue Sites
Site | Percent (%)∗ | |
---|---|---|
Eye11 | Staphylococcus spp. (coagulase −) | 0-11 |
Staphylococcus spp. (coagulase +) | 16-42 | |
Streptococcus spp. (beta hem) | 17-22 | |
Streptococcus spp. (alpha hem) | 2-9 | |
Streptococcus canis | 16.5 | |
Corynebacterium spp. | 3.5 | |
Enterococcus spp. | 0-5.6 | |
Escherichia coli | 4-17 | |
Proteus spp. | 0-2.6 | |
Proprionobacterium | 0-2.6 | |
Pseudomonas aeruginosas | 0-9.5 | |
Wound92 | No growth | 16 |
(n = 213) | Gram-positive | 53 |
Staphylococcus spp. (coagulase−) | 5 | |
Staphylococcus intermedius | 12 | |
Oral and other Streptococcus spp. | 6 | |
Streptococcus canis | 7 | |
Bacillus spp.† | 6 | |
Actinomyces spp. | 3 | |
Corynebacterium spp. | 2 | |
Gram-negative | 47 | |
Pasteurella multocida | 15 | |
Pasteurella canis | 5 | |
Other Pasteurellaceae | 2 | |
Enterobacteraceae | 7 | |
Vibrionaceae | 4 | |
Non–glucose fermenters | 16 | |
Urine105 | Escherichia coli | 45 |
(n = 8354) | Staphylococcus spp. total | 12 |
Proteus mirabilis | 6-12 | |
Klebsiella pneumoniae | 7-12 | |
Enterococcus spp.† | 6-9 | |
Enterococcus faecalis | (2-4) | |
Enterococcus faecium | (1-3) | |
Streptococcus spp. | 5 | |
Pseudomonas aeuruginosa | 3 | |
Mycoplasma spp. | 2-3 | |
Enterobacter spp. | 2-3 |
∗ Proportions are approximate.
Drug Preparations
Characteristics of topical ophthalmic antibacterial drugs to consider are the spectrum, and their lipid versus water solubility, with lipid solubility being more important if tissue penetration is of importance. Because of the ability to administer high concentrations of antimicrobials with topical administration, traditional classification of bacteriostatic versus bactericidal (fungal or viral -static or -cidal) may not be relevant to antiinfective drugs, and susceptibility data might underestimate topical efficacy. Ophthalmic drugs indicated for treatment of ocular infections are available as single or multiple antimicrobial agents (Table 8-2a and Table 8-2b). Generally, either gram-negative or gram-positive organisms are targeted using individual agents; mixed infections can be targeted with combination products or drugs characterized by a broad spectrum. Whereas few drugs are approved for use in animals, multiple human products are commercially available. Others can be compounded, which might include “fortification” of commercial products. However, the nuances of ocular preparations mandate that extreme caution be taken when formulating or modifying a product intended for topical ocular therapy (see Chapter 23).11
Table 8-2b Subconjunctival Doses of Drugs Also Used Systemically
Drug | Dose (mg) | Target |
---|---|---|
Bacitracin | 5000-10,000 U | Gram-positive |
Clindamycin | 15 to 50 | Gram-positive |
Erythromycin | 100 | Gram-positive |
Amikacin | 25-100 | Gram-negative |
Gentamicin | 10 to 20 | Gram-negative |
Polymyxin B | 100000 U | Gram-negative |
Streptomycin | 40-100 | Gram-negative |
Neomycin | 100-500 | Gram-negative>positive |
Penicillin | 500,000-1,000,000U | Gram-positive>gram-negative |
Ampicillin | 50-150 | Mixed |
Carbenicillin | 100-250 | Mixed |
Cefazolin | 50-100 | Mixed |
Ceftazidime | 100 | Mixed |
Ticarcillin | 1000 | Mixed |
Chloramphenicol | 40-100 | Mixed |
Drugs that target gram-negative organisms include the water-soluble, weakly basic aminoglycosides. At the high concentrations achieved topically, they are generally also effective against Staphylococcus spp. and include tobramycin (0.3%; drug of choice for treatment of Pseudomonas spp.), gentamicin (available with the glucocorticoid betamethasone), and neomycin (less effective toward Pseudomonas spp. and generally available only in combination with other antimicrobials). Polymyxin B, whose systemic use is precluded by nephrotoxicity, is a water-soluble drug, characterized by limited intraocular distribution. The fluoroquinolones also target gram-negative organisms, including Pseuodmonas spp. as well as Staphyloccoccus spp. In contrast to the aminoglycosides, the fluoroquinolones are lipid soluble. Drugs include ciprofloxacin, and ofloxacin and its L-isomer, levofloxacin. Systemic fluoroquinolones have been associated with retinal degeneration in cats (see Chapter 7). The adversity reflects accumulation due to a missing transport pump in the blood-retinal barrier (personal communication, Katrina Mealey, Washington State University). It is not clear if the adversity may occur with topical use, but prudence suggests that safety not be assumed.13 Drugs that target gram-positive organisms include the lipid-soluble erythromycin (0.5%; bacteriostatic), whose efficacy is limited by resistance, and the water-soluble bacitracin, which, like polymyxin B, is most known for its nephrotoxicity associated with systemic therapy. An example of a triple-antibiotic combination is one containing neomycin, polymyxin B, and bacitracin (see Table 8-2a). Broad-spectrum topical antimicrobials include chloramphenicol (prohibited for use in food animals), tetracyclines (drugs of choice for ocular Mycoplasma or Chlamydia), and the sulfonamides. Each is lipid soluble. Whitley11 has described a preparation of vancomycin (3.1%) and cefazolin (3.3%) compounded from injectable products., although the combination might be improved by replacing cefazolin with a drug with a broader gram-negative spectrum.
Topical antifungal are limited and include the polyene natamycin. Its spectrum includes all fungal agents except dermatophytes. The imidazoles must be compounded from intravenous solutions (i.e., miconazole, fluconazole, and itraconazole). Their spectrum includes opportunistic, dimorphic fungi and dermatophytes.
Treatment of Selected Infections
Use of systemic antimicrobials for treatment of ocular infections is complicated by poor drug penetration. Therefore topical therapy is indicated for external ocular infections, and subconjunctival administration is recommended for serious corneal or anterior chamber infections. For bacterial endophthalmitis, intravitreal antimicrobial therapy is indicated, although extreme caution is indicated for this route.13 Treatment of intraocular infections can be supported with systemic therapy of lipid-soluble drugs that can achieve bactericidal concentrations. In addition to antibacterials, collagenase inhibitors such as N-acetylcysteine, sodium citrate, bacitracin, and tetracycline compounds have been recommended for treatment of corneal infections.
Treatment with tetracycline is indicated for control of Mycoplasma and Chlamydia spp. Cats may develop hypersensitivity manifested as acute conjunctivitis with topical treatment.14 Oral therapy with tetracycline (5 mg/kg bid) stops shedding within 6 days. Azithromycin starting at 7 to 10 mg/kg qd for 2 weeks, followed by 5 mg/kg qd for 1 week, followed by 5 mg/kg qod for 14 days has been advocated, although the need for the complicated dosing regimen or the long duration is not clear. The presence of sneezing may indicate infection with feline calicivirus (FCV), for which supportive therapy is indicated. Conjunctivitis, often accompanied by ulcerative keratitis, can be a manifestation of feline herpesvirus (FHV-1), In addition to supportive therapy, topical preparations are available for its treatment (see also Chapter 10).15 Antivirals include vidarabine, trifluridine, and idoxuridine (the latter must be compounded). These are generally virustatic, requiring frequent application (every 1 to 2 hours is preferred, but every 4 to 6 hours is acceptable).
Systemic treatment of experimentally induced Chlamydia psittaci with 19 days of amoxicillin–clavulanic acid (12.5 to 25 mg/kg bid) has been compared with doxycycline (10 to 15 mg/kg qd; positive control) in 5-month-old cats (n = 24; 8 per group) using a randomized, placebo-controlled, blinded design.15a Outcome was based on clinical scoring, including respiratory signs or corneal changes; other clinical signs were not described. Both treatments were associated with rapid clinical improvement and reduced chlamydial isolation, with amoxicillin–clavulanic acid being associated with less isolation. However, five of eight of the cats treated with amoxicillin–clavulanic acid, but no doxycycline-treated cats, became positive 3 weeks after treatment. These cats became negative again with 4 weeks of retreatment with amoxicillin–clavulanic acid and remained negative 6 months later.
Systemic therapy was described in cats experimentally infected with C. felis. Once the organisms was detected, cats were treated with azithromycin (10 mg/kg qd for 3 days followed by twice weekly) (n = 9; two untreated negative controls, two doxycycline-treated positive controls [10-15 mg/kg qd]).16 At the end of the 21-day treatment period, untreated control cats were also treated. Despite an initial response to treatment (negative isolation to day 14), infection was eradicated in only one of five cats treated with azithromycin compared with both cats receiving doxycycline. However, it is not clear if the dosing regimen for azithromycin resulted in effective drug concentrations.
Otic Infections
Otitis Externa
Pathophysiology
Inflammation of the ear canal and the proximal pinna affects up to 20% of dogs, whereas fewer (up to 6% of) cats are affected.17 A number of causes can be identified in otitis externa, and their resolution is paramount to successful therapy. Possible causes include foreign bodies, allergies, parasites (e.g., mites, chiggers), skin disorders of a keratinous or sebaceous origin, and autoimmune disorders. Structural characteristics of the ear also can predispose the animal to otitis externa, including pendulous ears, higher number of ceruminous glands, the vertical and horizontal paths of the canal, hair in the ear canal, stenotic ear canals, or neoplasm. Environmental factors also can contribute to the difficulty in treating otitis externa, including external conditions that perpetuate excessive moisture (e.g., humidity, bathing) or heat or irritants (e.g., irritating medicaments or shampoos). The presence of yeast or bacteria that are part of the normal flora perpetuates the inflammatory process, and, with time, the inflammatory process itself will cause proliferative changes that complicate therapy. One of the more important predisposing factors to otitis externa is inappropriate treatment, including undertreatment and overtreatment.17 Ultimately, in some animals surgical treatment will be indicated (Figure 8-2).
Microbial Targets
Bacterial organisms found in normal ears include S. epidermidis and Staphylococcus intermedius (Staphylococcus pseudintermedius; see Chapter 6) and Micrococcus spp. Coliforms are found less commonly. Infection usually can be distinguished from colonization by the presence of large numbers of organisms, particularly if the culture is pure. Infection also is indicated by the presence of inflammation and phagocytized bacteria. Inflammation reflects, in part, the breakdown of fatty materials by organisms into irritating by-products. S. intermedius is the most common organism (30% to 50%) associated with otitis externa, followed by Pseudomonas aeruginosa, Proteus spp., Streptococcus spp., E. coli, and Corynebacterium spp. The infecting organism also appears to be time dependent in that acute otitis is generally associated with Staphylococcus, whereas chronic otitis more commonly involves Pseudomonas spp. This may refelect, however response to chronic antimicrobial therapy.
Petersen18 reported on the frequency and susceptibility of S. intermedius and P. aeruginosa in canine ear samples (n = 553) submitted to a state diagnostic laboratory between 1992 and 1997. Sampling methods varied. S. intermedius was isolated from 50% of ear samples but was the sole isolate in only 32% of the samples positive for S. intermedius. P. aeruginosa was isolated from 28% of ear cultures and was the sole isolate in 33% of P. aeruginosa samples.
The susceptibility of both S. intermedius and P. aeruginosa isolated from canine skin and ears appears to be changing across time (Table 8-3). A number of investigators have described their susceptibility. A review of the data suggests that the incidence of methicillin resistance is increasing, with a decrease in susceptibility of Staphylococcus spp. in general emerging in isolates collected during the period between 2003 to 2006. Morris19 focused on the susceptibility of methicillin-resistant isolates, including both coagulase-positive (S. aureus, and S. intermedius), as well as Staphylococcus schleiferi (including both coagulase-negative and positive subspecies; see also the discussion of Pyoderma). Of the total number of resistant isolates found in skin or ear, 28% of the methicillin-resistant S. aureus (MRSA), 40% of the methicillin-resistant S. intermedius (MRSI), and 33% of the methicillin-resistant Staphylococcus schleiferi (MRSS; presumed to be coagulase-positive subspecies schleiferi) were in the skin, and 5% MRSA, 26% MRSI, and 47% MRSS were located in the ear. Rubin20 described the susceptibility of 106 canine P. aeurginosa isolates cultured from soft tissue infections, including otitis externa and interna (see Table 8-3). No information was available regarding previous antimicrobial therapy in these animals. The limited amount of data precludes assessment across time; however, Pseudomonas is an organism recognized for its inherent resistance to multiple drugs, with an increasing tendency of resistance toward drugs to which it is normally considered susceptible (e.g., fluoroquinolones).
The combined data, particularly from Staphylococcus spp. isolates, demonstrate the limited susceptibility of these organisms to drugs most often used systemically or topically to treat organisms infecting the skin or ear. Culture and susceptibility data might increasingly become the basis for drug selection and dose design. Although the applicability of culture and susceptibility testing to the topical treatment of otitis externa may be controversial, the incidence of resistance is sufficient to justify culturing even in the earliest stages of infection, particularly in those patients at risk for recurrence, such that emergent resistance can be detected. Susceptibility testing is clearly recommended if gram-negative organisms are expected or in the face of severe or chronic otitis, failed antimicrobial therapy, and the presence of inflammatory cells. Testing is also recommended if systemic therapy is anticipated. Care should be taken to appropriately prepare and collect cultures from infected ears. Frustratingly, the accuracy of susceptibility testing may be questioned simply because of differences in laboratories. Schick21 compared the results of cultures simultaneously submitted from dogs with otitis. Swabs were rubbed together to ensure similar samplings. Labs agreed regarding the presence of Pseudomonas spp. only 83% of the time. Susceptibility of the isolates to amikacin varied in 13 of 16 samples, for gentamicin 10 of 16, and for enrofloxacin 9 of 16.
Antimicrobial and Adjuvant Options
The public health implications of treating methicillin-resistant Staphylococcus spp. in dogs or cats are addressed in the discussion of Pyoderma. Because successful treatment of otitis externa is critically dependent on proper cleaning, it is difficult to separate antimicrobial therapy from adjuvant therapy. The goals of therapy for otitis externa are to identify and resolve the primary factors, reduce inflammation, and control or eradicate infection. Sedation or anesthesia may be necessary for proper cleansing. Cleansing should remove irritating oils, waxes, and other debris that might serve as a nidus of infection by providing a microenvironment favorable to the growth of microorganisms. Ear cleaning should be less aggressive in the presence of severely swollen or proliferative canals; in such patients initial therapy might begin with antiinflammatory doses of glucocorticoids and antimicrobials, both systemically and topically.
Ceruminolytics contain various surface active agents or emulsifiers (dioctyl sodium sulfosuccinate, carbamide peroxide, squalene, propylene glycol, glycerin, oil) that dissolve wax accumulation and associated debris. They should be used as an initial flush. Less messy water-soluble agents (dioctyl sodium sulfosuccinate, propylene glycol) are often preferred.22 Carbamide peroxide, a common ingredient of human preparations that is seldom found in veterinary preparations, releases nascent oxygen, causing a bubbling action that softens and removes debris. Combination products may contain drying agents such as isopropyl alcohol, silicone dioxide, and alpha hydroxy acids (lactic, malic, or salicylic acid). In addition to their drying effects, these materials may also have mild antimicrobial effects.22
Flushing solutions facilitate removal of debris. Although water and saline are the safest, some products contain germicidal ingredients. Chlorhexidine can be diluted to a 0.05% solution and used topically. Although ototoxicity should lead to cautious use in the presence of a perforated eardrum, one study failed to provide evidence of damage after 21 days of therapy in dogs with experimentally traumatized membranes; the power of the study to detect a significant difference is not stated.22 Povidone–iodine at 0.1% to 1% can provide bactericidal activity in flushing solutions, although it can cause contact irritation. Because of ototoxicity, solutions with less than 0.5% iodine are preferred if the eardrum is ruptured. Iodine might also be administered as a polyhydroxidine complex (Xenodine). Because it contains 0.5% of titratable iodine, it is less irritating and provides a longer duration of activity. Efficacy against Pseudomonas spp. has been established with this product. It must be used in an aqueous environment and therefore should be used within 2 hours after cleaning to maximize its effects.22 Acetic acid is a relatively inexpensive agent, available as white or brown vinegar in a 5% solution. Antimicrobial effects occur because of direct damage as well as acidification of the local environment. The acidic pH may also facilitate removal of necrotic debris. Pseudomonas spp. succumbs to a 2% solution within 1 minute of contact,22 whereas a 5% solution is effective against Staphylococcus spp. Streptococcus spp., E. coli, and Proteus spp. The preparation is irritating at 2% to 5% concentrations, however, and inhibition of wound healing and ototoxicity may occur at concentrations of 2.5%. Dilutions of 1:1, 1:2, or 1:3 in water daily or every other day have been recommended.
Topical antimicrobial treatment of otitis externa is generally indicated regardless of whether systemic therapy is implemented. Topical products intended specifically for treatment of otitis externa (Tables 8-4 and 8-5) generally contain various drugs intended to target bacteria, yeast, and inflammation. Vehicles are designed to maximize drug solubility (only dissolved drug will passively diffuse) but must also allow drug movement from the vehicle into tissues. Antibacterial agents often include products that are associated with severe systemic toxicity when given orally or parenterally; topical administration generally is not associated with systemic adversities.
On the basis of a MEDLINE search and review of the literature in human medicine, the ototopic use of antimicrobials does not appear to be associated with an increased risk of antimicrobial resistance.23 However, the relevance to canine or feline ear, for which the pathophysiology is substantially different, is not clear. Ototoxicity is, however, likely for many antimicrobial drugs when administered in the ear canal, especially in the presence of a perforated tympanic membrane (see later discussion).
Topical antimicrobials generally are selected on the basis of cytology and potentially Gram staining. A number of products are commercially available (see Table 8-4). For selected drugs, ophthalmic (directly or diluted) or injectable (diluted) products may also be used. Vehicles used in topical products represent a balance between drug solubility and drug delivery.It may be necessary for vehicles to be pH balanced to maximize solubility. Demulcents (polyethylene or propylene glycol or glycerin) act to suspend non–water-soluble drugs; the glycol agents may irritate an already irritated ear. DMSO is included in some otic preparations as a vehicle, although it also has significant antiinflammatory effects. The agent is very hygroscopic and as such is an effective carrier agent for other drugs included in an otic preparation. In addition, DMSO provides mild antibacterial and antifungal actions as well as antiinflammatory and antifibroplastic effects. Other vehicles used to administer drugs useful for otic disorders include solutions, lotions, ointments, and other oil-based products. Occlusive oils may be undesirable for exudative lesions. Ointments and oil-based products can, however, be used in cases of chronic otitis externa that are dry. Generally, topical preparations should be applied twice daily. A number of home remedies have been recommended for treatment of otitis externa (see Table 8-5). Caution is recommended when making new preparations or modifying old preparations. A number of drug interactions between drugs and their vehicles can inhibit the efficacy of any of the drugs. In addition, drugs must be dissolved to be effective, and the addition of solid drugs (i.e., as powders or crushed tablets) to ointment vehicles (e.g., petrolatum jelly) is likely to yield an occlusive but minimally effective agent. Some acceptable modifications of commercially available products are noted in Table 8-5.
Note that modification of a product may alter the stability, and a shelf-life of 1 week or less might be prudent unless data exist to support a longer beyond-use date. Several in-practice compounded preparations have been described elsewhere.24
Examples of antibacterials used in otic preparations to target gram-negative organisms include the aminoglycosides gentamicin (0.3%), tobramycin (0.3%), and neomycin (0.33%) and the cell membrane–active agents colistin (0.3%) and polymyxin B (5000 IU/mL). Topical aminoglycosides also target Staphylococcus spp. whereas systemically, amikacin is more effective toward Pseudomonas spp., and gentamicin is more effective toward Staphylococcus spp., systemic use of an aminoglycoside as sole therapy for treatment of Staphylococcus spp. is discouraged. An otic preparation for amikacin can be compounded from the commercially available injectable product by adding 30 to 50 mg/mL to sterile saline or Tris-EDTA. Because aminoglycosides are more effective in an alkaline environment, they should be used either before or 1 or more hours after acidifying agents. Silver sulfadiazine (0.5%) is an ointment approved for humans to prevent or treat infection in burn patients. Its spectrum also includes Pseudomonas spp. However, the product, as approved in humans, may cause skin irritation. Silver sulfadiazine (1%) is available in commercial products combined with enrofloxacin (0.5%) or as a micronized powder (e.g., www.spectrumrx.com) that can be reconstituted in sterile water to a 0.5% to 1% solution (5 and 10 mg/mL, respectively). An otic preparation containing orbafloxacin and posaconazole as an antifungal drug with mometasone as an antiinflammatory has recently been approved for dogs (Table 8-4). A number of other antimicrobials have been administered topically, including the narrow-spectrum fusidic acid for treatment of Staphylococcus spp., antipseudomonadals such as colistin or polymyxin B (10,000 U/mL of Neosporin GU,24 fluoroquinolones (discussed later), and ticarcillin (which also targets Staphylococcus spp.). Ciprofloxacin and ofloxacin are available as human otic preparations. Ciprofloxacin is generally more potent than enrofloxacin against Pseudomonas spp.
Trothmethamineethylenediamine-tetraacetate (EDTA, Tris-EDTA, or commercial Triz/EDTA) is a topically applied compound that chelates cations, thus rendering the cell wall and membrane of both gram-positive and gram-negative organisms more permeable to antimicrobials. Penetration of antibacterials subsequently is facilitated. Treatment should be timed such that microorganisms are exposed to Tris-EDTA before peak concentrations of antimicrobials reach the site of infection. Direct addition of antibiotics such as gentamicin to the buffer solution may be less desirable than pretreatment with Tris-EDTA, followed later by antimicrobials (to allow time for chelation of cations). Because cations are also removed from the lipopolysaccharide covering, EDTA may be particularly effective for treatment of gram-negative organisms. Aminoglycosides in particular are facilitated because cations that otherwise would repel positively charged drugs such as aminoglycosides are removed. Synergisim has been demonstrated with Tris–EDTA and aminoglycosides (amikacin and neomycin) against S. intermedius, Proteus mirabilis, P. aeruginosa, and E. coli. Combination solutions appear to be stable for at least 3 months.25
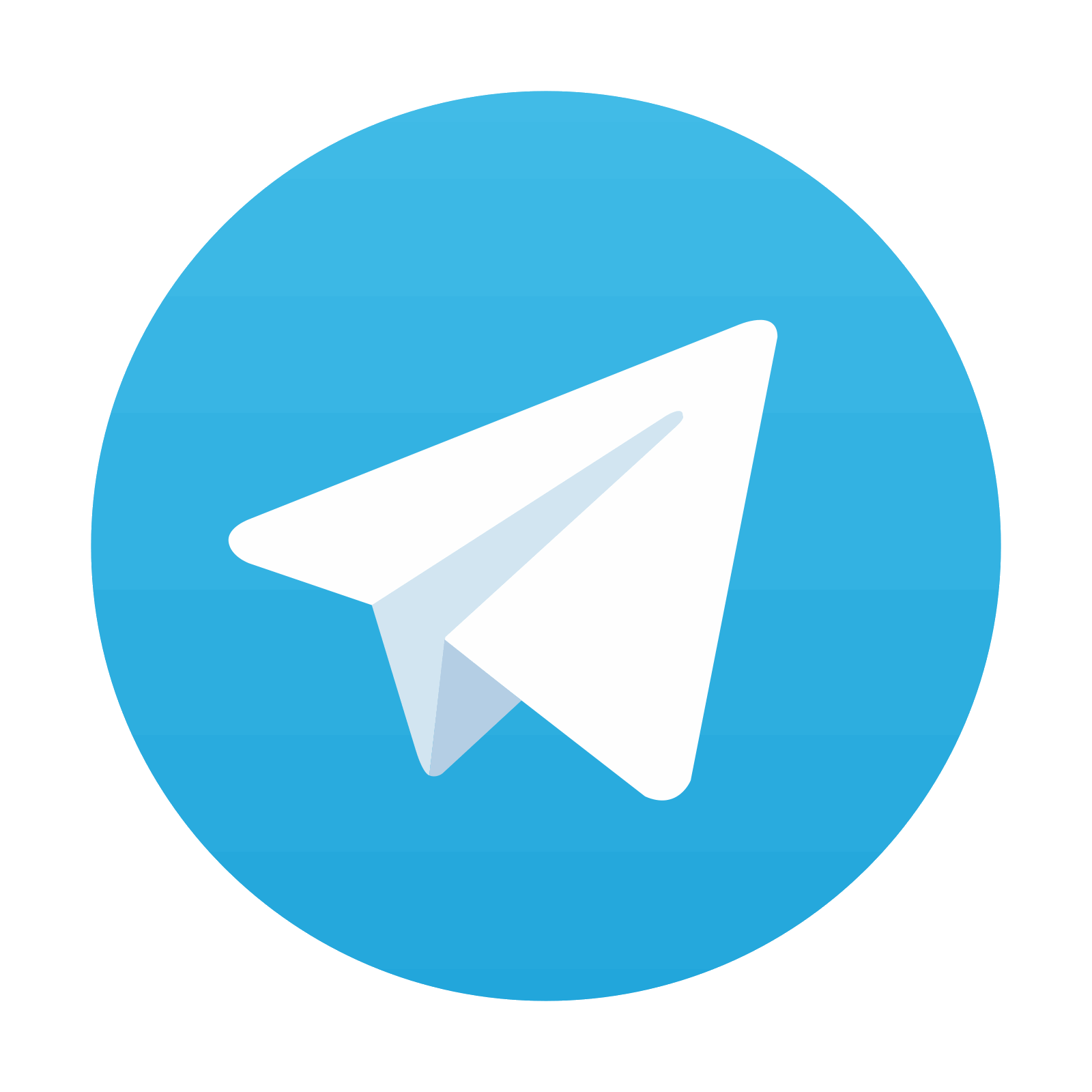
Stay updated, free articles. Join our Telegram channel
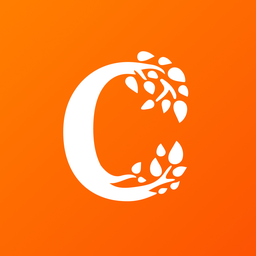
Full access? Get Clinical Tree
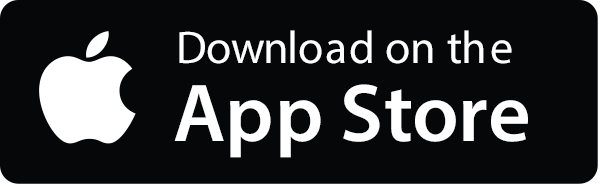
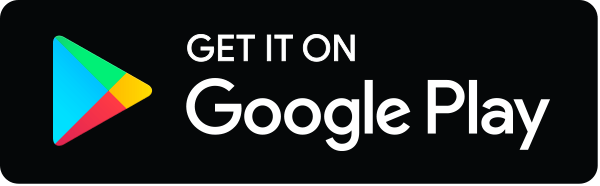
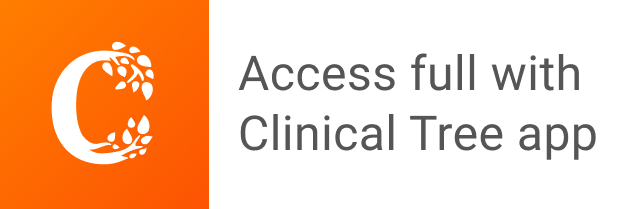