, Monika Hassel2 and Maura Grealy3
(1)
Centre of Organismal Studies, University of Heidelberg, Heidelberg, Germany
(2)
Spezielle Zoologie, Universität Marburg FB Biologie, Marburg, Germany
(3)
Pharmacology and Therapeutics, National University of Ireland Galway, Galway, Ireland
The human central nervous system is considered the most complex organ a living being has ever developed. As measured by its size it certainly is the most complex system in our field of experience. Based on weight, on the total DNA content, and on counting the number of cells in a manageable defined section and subsequent statistical projection, our brain has been estimated to encompass 100 billion (1011) nerve cells and 1,000 billions (1012) of glial cells and cells of the immune system (known as microglia). Each nerve cell is connected with >1,000 others. A spinal motor neuron with a relatively modest number of dendrites receives about 10,000 contacts. The dendritic tree of a Purkinje cell in the cerebellum is much larger and receives approximately 150,000 contacts. The total length of nerve fibres has been estimated to surpass 500,000 km.
To describe and understand the development of the nervous system is among the most demanding task of the contemporary biological science. In this chapter we confine ourselves to a general outline of the development of the vertebrate brain (with an eye to insects). We view the formation of the neural tube; that is the primordium of the central nervous system (CNS), we track migrating cells which give rise to the peripheral nervous system (PNS) including the enteric nervous system (ENS). We observe how neurons form their information-transmitting processes and seek synaptic contact to other cells. We pose the question how this complex order is organized.
16.1 Morphological Developmental History of the Nervous System
16.1.1 The Central Nervous System Arises From the Neural Tube, Whereas The Peripheral Nervous System Is Established by Neural Crest Cells
(a)
The central nervous system, CNS. Recall that in vertebrate embryos, the central nervous system forms along the dorsal midline of the body axis. The morphological formation of the nervous system begins during gastrulation, when dorsal mesoderm (chordamesoderm) moves into contact with overlying ectoderm. The dorsal mesoderm emits signals which induce the overlying ectoderm to adopt a neural fate (see below). The induced neural plate forms neural folds (Fig. 16.1), the folds form the neural tube, and the neural tube forms brain and spinal cord (Figs. 16.1, 16.2, 16.3 and 16.4). The peripheral nervous system, including the neuronal network of the gut is still missing from the list. The peripheral nervous system derives from emigrated neural crest cells (Sect. 16.4).
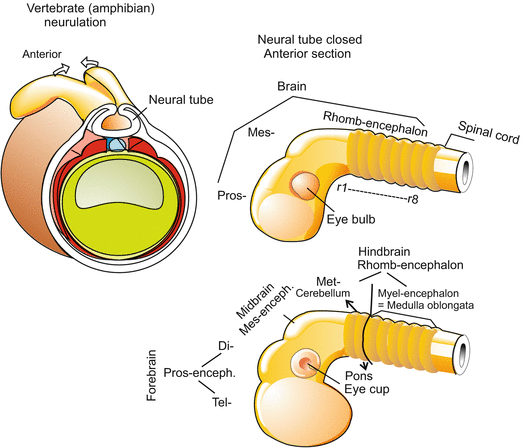
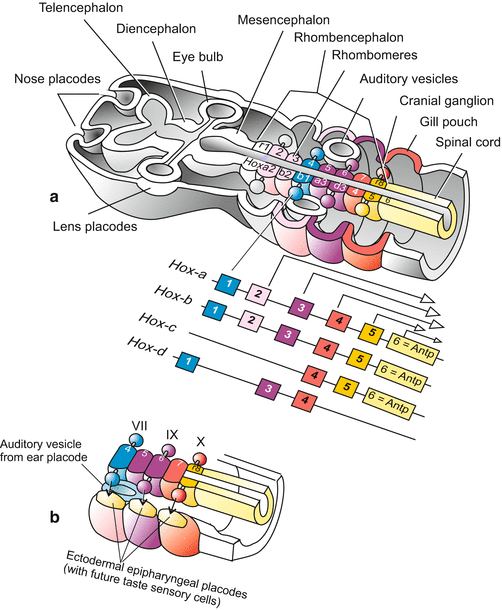
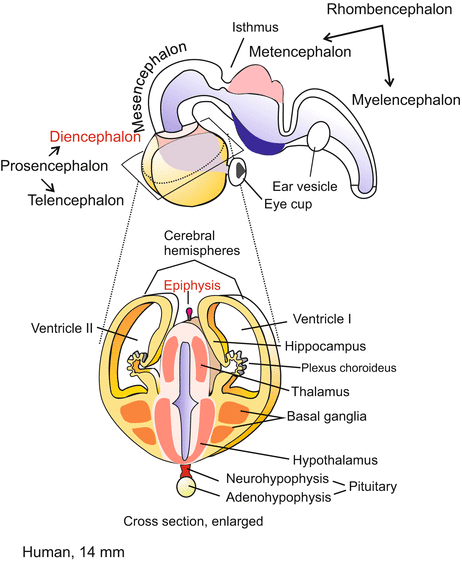
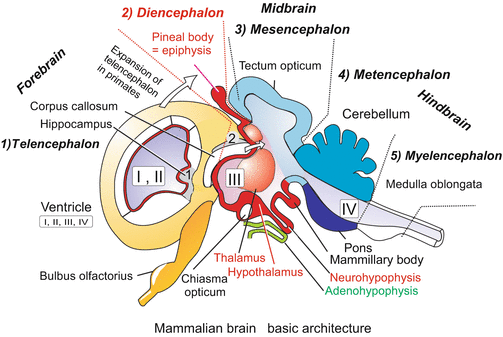
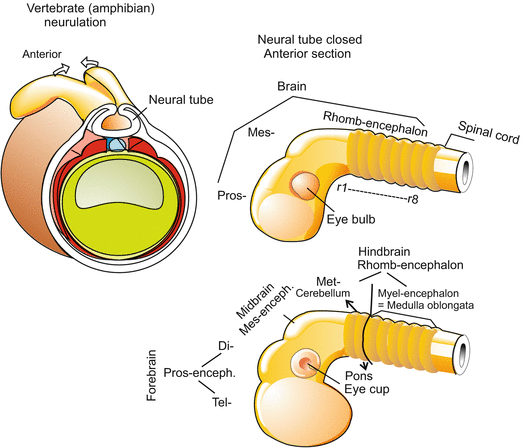
Fig. 16.1
Formation of the central nervous system exemplified by the amphibian embryo. Proceeding from anterior to posterior first the three-part brain with prosencephalon, mesencephalon and rhombencephalon is formed. Later, the three-part brain is further subdivided into five sections. The prosencephalon gives rise to the telencephalon and diencephalon, the mesencephalon remains single-section, the rhombencephalon subdivides into metencephalon and myelencephalon
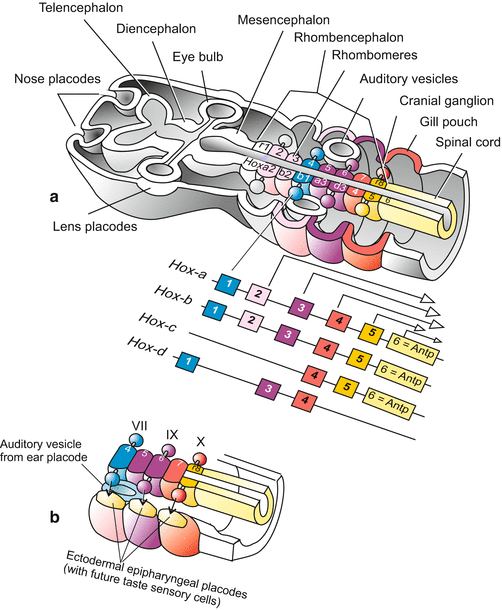
Fig. 16.2
(a, b) (a) Development of the brain and adjacent sensory organs in a generalized mammal. The posterior part of the brain, the rhombencephalon, is subdivided into repeating units called rhombomeres or neuromeres. Cranial ganglia are associated with the even-numbered rhombomeres (r2–r8). While the eye vesicle arises from the posterior forebrain (diencephalon) by evagination, the eye lens, the nasal epithelium and the inner ear arise from placodes, thickenings of the epidermis that are invaginated. In addition, the expression pattern of some Hox genes [after Alexander et al. (2009)] is shown. This pattern helps to identify and demarcate rhombomeres. (b) Excerpt, showing in addition the epipharyngeal (epibranchial) placodes, which give rise to the future taste receptor cells. They are innervated by nerve fibres (arrows) emanating from the cranial ganglia (= cranial nerves) VII (nervus facialis), IX (n. glossopharyngeus) and X (n. vagus). The otic vesicle gives rise to the inner ear and is innervated by the cranial nerve VIII (n. vestibuloocularis) which emanates from the myelencephalon
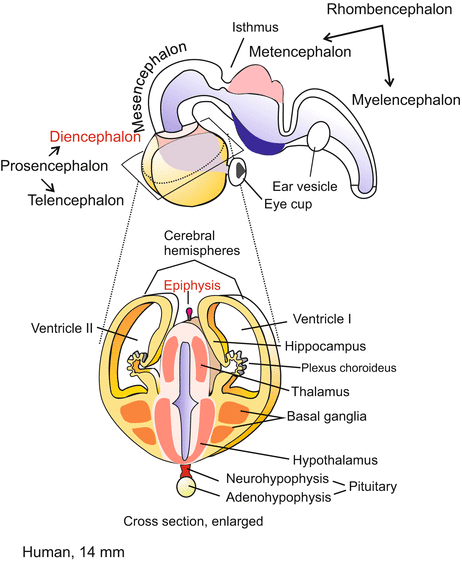
Fig. 16.3
Early stage human brain. The section shown below shows the diencephalon in the centre and left and right from it the hemispheres of the paired, caudally expanding telencephalon (cerebrum). Eventually the paired hemispheres enclose the diencephalon completely
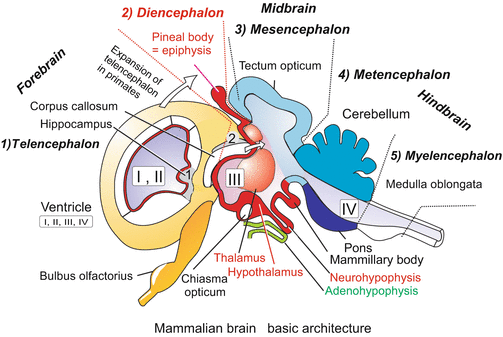
Fig. 16.4
Advanced mammalian brain. Median cut, but in addition one of the hemispheres is cut by a separate paramedian incision. In humans the hemispheres expand in posterior direction and eventually cover the di- and mesencephalon completely and the cerebellum partially. Correspondingly the fibre bundle connecting the two hemispheres known as corpus callosum expand posteriorly. The grey fields 1 (hippocampus) and 2 are remnants of the ancient archaeopallium. The pineal body (epiphysis) is formed as an evagination of the dorsal midbrain. The pituitary gland is composed of two different parts: The neurohypophysis (posterior lobe) arises from an evagination of the ventral diencephalon (hypothalamus); the adenohypophysis arises from an evagination of the roof of the pharynx
(b)
The peripheral nervous system, PNS comprises
the dorsal root ganglia, also known as spinal ganglia,
the peripheral components of autonomic nervous system, that is the sympathetic system and the parasympathetic system, as far as the cell bodies with the cell’s nucleus (called perikarya) are located outside the CNS. Sympathetic neurons are found in the switching stations known as paravertebral ganglia along the sympathetic trunk (see Figs. 16.17 and 16.18) and in visceral ganglia; parasympathetic neurons are found close to, or within, the target organs (Fig. 16.17);
the autonomous nerve net of the gastro-intestinal tract. This part of the classic autonomic (vegetative) system is currently ranked as a separate nervous system and called ENS, enteric nervous system, but it belongs to the PNS.
16.1.2 The Brain of the Vertebrates First Divides into Three and then into Five Segments
It seems curious that most of our brain is initially a hollow cavity. In its anterior part, the neural tube balloons into three main vesicles: forebrain (prosencephalon), midbrain (mesencephalon), and hindbrain (rhombencephalon). In a second step, forebrain and hindbrain are subdivided into two parts each and we see the classic five parts of the textbook brain:
Prosencephalon
1.
Telencephalon (cerebral hemispheres, with the neocortex)
2.
Diencephalon
Mesencephalon; it is not further subdivided and remains
3.
Mesencephalon
Rhombencephalon
4.
Metencephalon (cerebellum)
5.
Myelencephalon (medulla oblongata)
In humans the following principal functions are assigned to these regions:
Telencephalon. Evaluation of the information supplied by the sensory organs of smell and taste, by the inner ear with its semicircular canals and balance system, evaluation of acoustic and visual information (in lower vertebrates performed by the mesencephalon). Generation of associations, short-term, working and long-term memory, attention.
Although in humans the largest part of the telencephalon comprises the evolutionarily young neocortex (Fig. 16.5), in its ventral part the telencephalon also harbours old core regions called “nuclei”. In the traditional terminology of neuroanatomists “nuclei” are areas of “gray matter” that contain numerous densely arranged cell bodies with cell nuclei (perikarya), while the “white matter” preferentially consists of nerve fibres (nerve tracts). The phylogenetically ancient structures include the rhinencephalon (olfactory brain) and a series of core regions which in the traditional nomemclature are called basal ganglia (with striatum and pallidum) classified as part of the limbic system. Some often mentioned structures are shown in Fig. 16.5. Of particular significance is the paired hippocampus (also known as Ammon’s horn), a structure of the old cortex which is displaced by the expanding neocortex to a lateral-ventral position underneath the temporal lobe. The hippocampus has a leading role in the selection and transfer of memory contents into the long-term memory. The limbic system is responsible for the generation of emotional experiences. Its components are connected by neuronal fibres with the hypothalamus of the diencephalon.
Fig. 16.5
(a, b, a1–a3) Enlargement of the neocortex in humans and limbic system. Together with the neocortex also the corpus callosum expands. Through insertion of new cortical areas the evolutionary ancient structures of the limbic system (LiS) are relatively displaced (the thalmus depicted as a structure of reference does not count among the LiS). Here mapped components of the limbic system are the gyrus cinguli, basal ganglia of the telencephalon (with septum and nucleus accumbens), amygdala, hippocampus, the fibre bundle of the fornix, which establishes an anatomical connection between the basal ganglia, the hippocampus and the hypothalamus, the central instance of vegetative functions. (b1-2) The pictures b1 and b2 refer to the corresponding structures of the adult brain and show in addition the mammillary bodies (corpora mamillaria), which also are attributed to the LiS. The functions of emotion and memory are ascribed to the limbic system. The hippocampus plays a leading role in deciding which content of the memory is transferred into the long-term memory
Diencephalon. The diencephalon is the origin of the eye vesicles (Figs. 16.1 and 16.2). It is subdivided into
Thalamus: Relay centre for optic and acoustic tracts; gateway for sensory input passing from the spinal cord to the cerebellar hemispheres. The mammalian metathalamus encompasses the lateral geniculate nucleus (corpus geniculatum laterale), the switching station for visual information, and the corpus geniculatum mediale, the switching station of the acoustic tract.
Epithalamus and hypothalamus: Supreme commander of basic vegetative functions, control centre of inner homeostasis, sleep, alertness. Connects the nervous and hormonal systems. To prepare for the latter task, the
the ventral hypothalamus extends the infundibulum which will form the posterior lobe of the pituitary, the neurohypophysis.
The pituitary gland is completed by a structure which emerges not from the brain but from the roof of the pharyngeal cavity. By a local evagination, the roof forms Rathke’s pouch, which transforms into the anterior lobe of the pituitary gland, the adenohypophysis.
Mesencephalon. Forms in non-mammalian vertebrates the tectum opticum, the main centre of visual data processing. In mammals visual information is forwarded to the V1 region of the telencephalon via the changeover facility of the lateral geniculate nucleus (see Fig. 16.25), while the tectum opticum is merely a relay station for visual and acoustic reflexes and the acoustic tract.
Cerebellum. Main location where complex automatic movements are programmed and coordinated.
Myelencephalon. Reflex and control centre for vegetative functions, oscillators for breathing, control of blood circulation, reflexes for suction, swallowing, coughing.
16.1.3 Discussed Since the Days of Goethe: Is the Brain Subdivided into Segments, Like the Vertebral Column?
Since the days of Johann Wolfgang von Goethe (1749–1832) and Lorenz Oken (1779–1851) there has been continuing discussion whether or not the skull, and with it the brain, is subdivided into segments. Not only morphological constrictions but more so the expression patterns of Hox genes speak for themselves: yes the hindbrain is segmented.
The hindbrain is initially patterned into seven repetitive bulges, called rhombomeres (Figs. 16.2 and 16.3). The first rhombomere gives rise to the cerebellum. Each subsequent pair of rhombomeres contains a set of motor neurons and contributes fibres to one cranial nerve root. These are connected with the cranial ganglia which arise from neural crest cells. The first ganglia are attached to the even-numbered rhombomeres, whereas the odd-numbered rhombomeres are connected with ganglia only later.
With respect to the anterior brain regions, prosencephalon and mesencephalon, there are no good arguments in favour of an original segmental organization as in these regions transcription factors other than Hox-coded ones control development, and the anatomist does not find periodically repeating structures. Based on gene expression studies some scientists proposed the forebrain to be divided into six segment-like domains called prosomeres but this proposal was not agreed by the majority of scientists. It is not currently known how the internal subdivision of the forebrain comes about.
The brain is surrounded and supplemented by the main sensory organs needed for long-distance orientation and for complex behavioural control (Fig. 16.2).
16.1.4 The Subdivision of the Brain Is Controlled by Local Organizing Centres
As explained in the following chapter in the arising central nervous system diverse genes are expressed that code for transcription factors highly conserved in the animal kingdom, or code for soluble, secreted factors that act directly on the neighbourhood. An important role in the antero-posterior patterning of the brain is played by the isthmic organizer. Isthmus is the designation of a constriction between midbrain and hindbrain (Fig. 16.6). In the tube stage of the CNS at the posterior boundary of the future midbrain, and just anterior to the future isthmus, a strip of cells begins to produce the signalling molecules FGF-8 and WNT-1. These signals are later emitted by this organizing centre and control the regional specification of the brain on either side. To the anterior the centre induces midbrain, to the posterior it induces the rhombomere 1 to form the cerebellum. If a piece of the isthmic organizer is transplanted into a donor further caudally into the region of the myelencephalon a second midbrain is formed there. A similar result can be brought about by inserting a bead soaked with FGF-8. Probably more organizing centres emerge until the basic architecture of the brain is realized.
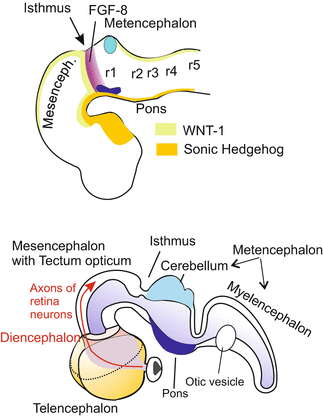
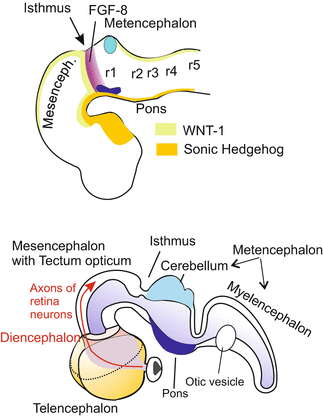
Fig. 16.6
Position of the isthmus organizer at the mid brain/hindbrain border and areas where some soluble signalling proteins are produced. Anterior and adjacent to the future isthmus a strip of cells starts expressing the signalling protein WNT-1 and a posterior strip starts expressing FGF-8 in a steep declining gradient. Both WNT-1 and FGF-8 have important functions in the establishment of the midbrain/hindbrain boundary. In addition, the boundary is determined by the activity of transcription factor genes such as Otx2 and Gbx2
16.2 Genetic Programming of the Nervous System
16.2.1 The Nervous System Arises From Cell Areas That Are Prepared for Their Task by Maternal Proneural Transcription Factors
The Programming of the Future Nervous System is a Multi-Step Process.
Even though we place special emphasis on the development of the mammalian and human nervous system, once more first the amphibian embryos have to act as a surrogate model. It has long been established that in the late blastula when the Spemann organizer commences operation this transmitter emits signals that give the cells of the animal hemisphere the option to become nerve cells. Such signals are represented by induction factors such as NOGGIN and CHORDIN which for their part neutralize the anti-neural factor BMP3/4. This anti-neural factor is needed to suppress neural development in the ventral embryo and to program the ventral ectoderm to become epidermis. A decades-old hypothesis implies the cells of the animal cap would have the autonomous tendency to become nerve cells but would initially be prevented from pursuing their propensity. This hypothesis was recently corroborated by molecular biological findings and experiments. Already in the egg cell maternally transcribed mRNA for proneural transcription factors is deposited, for example mRNA for the factor SOX3 which contains a DNA-binding HMG domain, and further factors providing competence for neural differentiation (Fig. 16.7).
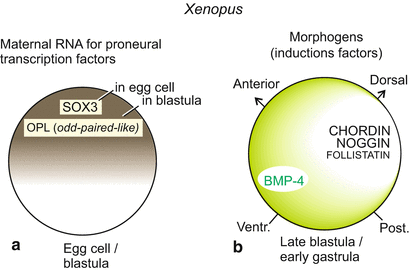
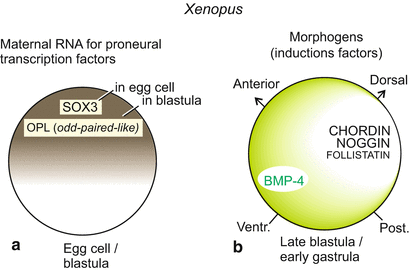
Fig. 16.7
Factors specifying the central nervous system. Factors deposited in the egg cell such as the maternal SOX3 initiate specification, supplemented by OPL subsequent to fertilization. SOX3 includes a DNA-binding domain known as HMG motif. OPL is a zinc finger protein homologous to the Odd-Paired factor of Drosophila. These transcription factors confer on the animal region the competence to become nerve tissue. In the ventral region of the embryo this competence is suppressed by BMP3/4, whereas the cells of the animal hemisphere are prepared to respond to neuralizing induction factors. These unveil the proneural competence. BMP3/4 is neutralised by CHORDIN and NOGGIN, which radiate from the organizer
Inherited from the mother, these factors can turn on expression of zygotic (embryo’s-own), nerve cell-specifying transcription factors in the cells of the animal hemisphere. Once more we learn that in the control of developmental processes one has to distinguish between
1.
extracellular factors that direct large cell associations, and
2.
transcription factors located inside the cells, and eventually within the nucleus, that control gene activities.
16.2.2 Induction Factors Switch on Further Proneural Selector Genes
Extracellular signals involved in the specification of the vertebrate nervous system have been revealed by various methods. Originally, explants from Xenopus blastulae (classic animal cap assay, Fig. 16.8) were treated with solutions that putatively contained factors stimulating nerve cell differentiation. Currently, embryonic stem cells of the mouse or humans are treated with sundry factors aiming at stimulating them to differentiate into nerve cells (Chap. 18). In such assays it is checked which type of nerve cell or glial cell arises from originally “naive” cells. Without going into the experimental details we summarize:
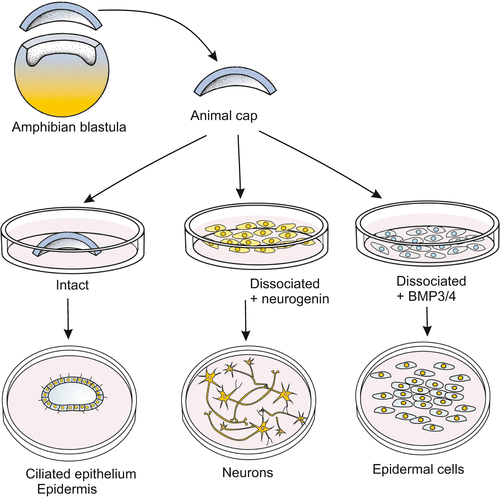
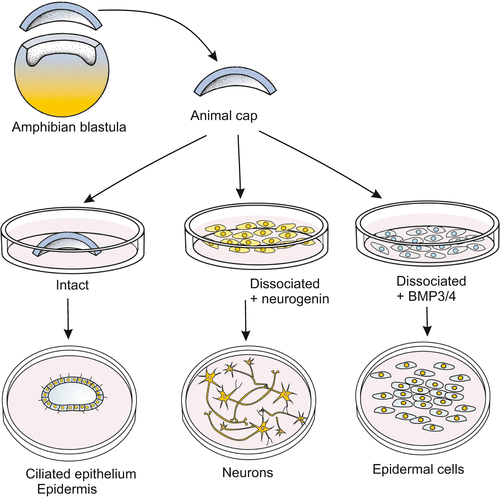
Fig. 16.8
Checking the effect of factors, for instance the anti-neuralizing action of BMP3/4, in the animal cap assay. In newts dissociation alone discloses the bias of animal cap cells to form nerve cells. The bias is suppressed by BMP3/4
Besides the already mentioned anti-BMP4 factors CHORDIN and NOGGIN also factors of the WNT family and the FGF family participate in uncovering the neuronal potency; supported by the non-protein factor retinoic acid RA.
FGF: Subsequent to treatment with anti-BMPs Xenopus ectoderm expresses FGF-4. This factor is needed to convert cells into nerve cells.
WNT: Already in hydrozoan polyps of the genera Hydra and Hydractinia the WNT-β-catenin system participates in neuronal differentiation. In vertebrates WNT molecules are at play when it comes to subdivide the central nervous system into brain and spinal cord.
Regionalization of the CNS along the longitudinal body axis (Fig. 16.9). This subdivision is prepared early during gastrulation. The WNT-8 signals emanating from a centre at the blastopore and spreading in the anterior direction favour specification of spinal cord. In the anterior region of the neurula WNT-8 molecules are bound and neutralized by the factors DICKKOPF and CERBERUS and this favours brain formation. Besides permissive (permitting) factors such as CHORDIN and NOGGIN also putative positive inducing signalling molecules appear to be involved in the switching on of nerve cell-specific genes as indicated by findings made in the chick embryo.
Fig. 16.9
Regionalization of the CNS in the neurula. Of particular significance are induction factors released from the notochord primordium and the prechordal mesoderm known as CERBERUS, DICKKOPF, CHORDIN, and NOGGIN. These initialize the subdivision of the central nervous system in forebrain, midbrain, hindbrain and spinal cord. In this function they are supported by further factors which are present in the form of gradients extending along the body axis from posterior to anterior such as WNT-8, WNT-3, WNT-11, FGF-4 and RA (Retinoic Acid)
16.2.3 Step-by-Step Further Proneural and Neurogenic Genes Coding for Transcription Factors Are Switched On
Proneural means: “in preparation of”, “in favour of nerve cells”; neurogenic means: “nerve cell generating”.
In the Xenopus embryo (and likewise in the fish embryo) the ontogenetic history of the nervous system starts with the maternal factor SOX3. Soon thereafter in the nuclei of the arising neural plate further proneural transcription factors such as NeuroD, neurogenin, Mash1, Math und NEX are found. They belong to the ATONAL group of transcription factors having a bHLH (basic-Helix-Loop-Helix) motif as DNA-binding domain. In Drosophila proneural genes are clustered on a chromosome forming the Achaete-scute-complex (As-C). In vertebrates homologues of the As-C-gene cluster (such as Asc1/lash) are only expressed in a subclass of neurons. Probably, there exist further such fate-determining genes.
Most currently identified genes/factors are responsible for the development of the nervous system throughout the entire animal kingdom, starting with the cnidarians, the most ancient, still living animal phylum possessing a nervous system. In vertebrates all these factors emerge in changing combinations in the entire developing nervous system. They stimulate stem cells to become nerve cells, and nerve cells to differentiate into a certain subtype, including glial subtypes.
The finding that in the entire animal kingdom homologous (orthologous) genes are at play when it comes to program the nervous system is, in retrospect, not surprising. Nerve cells share many features: they form thin processes for receiving and transmitting chemical and electrical signals; their cell membranes are equipped with ion channels of various kinds; they generate and release transmitters. Future research has to figure out in detail where all the proneural and neurogenic genes intervene and make their contribution.
16.2.4 The Future Nervous System Is Separated From the Prospective Epidermis by the Notch/Delta Mechanism of Decision Making
At the margin of the neural plate, but also within the neural plate (as outlined below), the primary neurons must segregate from their surroundings (Fig. 16.10). This happens by means of the Notch/Delta system, whose function in mediating lateral inhibition was introduced in Fig. 10.1. In principle a future neuroblast presents the Notch receptor of its neighbours with a stronger inhibitory Delta signal than the Delta signal that the neighbours can respond with. The situation is inverse with receptors. The neuroblast with superior power to suppress neighbours becomes itself more and more insensitive to suppressing influences by its neighbours because it withdraws its own Notch receptors. With time inhibition becomes more and more unidirectional and eventually the neuroblast is definitively the winner, adjacent cells are the losers.
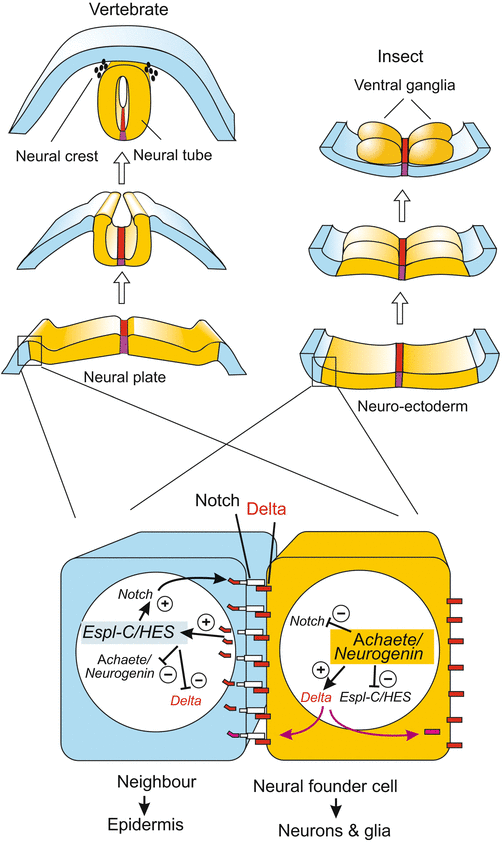
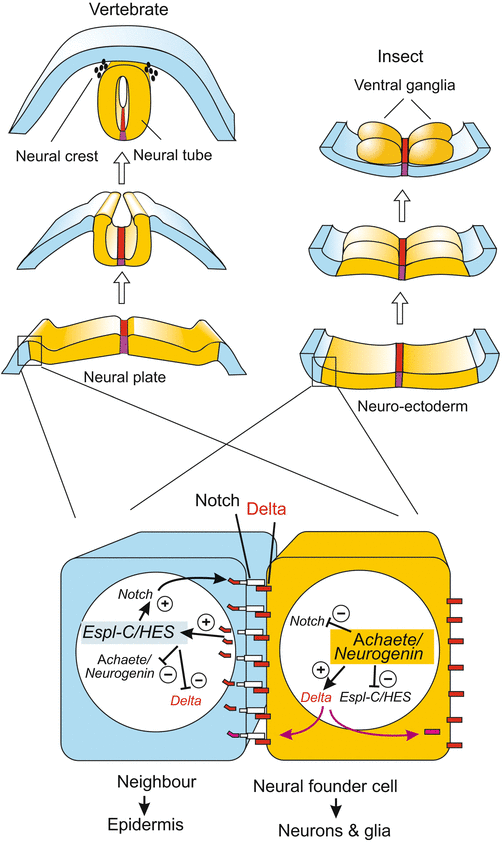
Fig. 16.10
Role of the NOTCH/DELTA system and of proneural genes in alternative fate decisions along the margin of the future CNS. The decision neuroblast versus epithelial cell, and neuron versus glial cell is made in originally quite similar cells. Both produce the inhibitory DELTA signal that suppresses neuronal differentiation, and both initially had receptors of the NOTCH class to receive DELTA signal. Thanks to a slight initial advantage the future neuroblast dominates more and more by producing more and more DELTA while degrading its own NOTCH receptors and thus being protected against the DELTA presented by its neighbour. Epidermis: In the left, blue cell a presenilin protease cleaves off the intracellular domain of NOTCH, called NICD. NICD enters the nucleus and becomes part of a transcription factor complex that switches on an epidermis-specific gene cluster. In Drosophila this cluster is known as Enhancer of split, in vertebrates as HES. The neuroblast (yellow) on the other hand can switch on neurogenic genes unhampered. These are in Drosophila genes of the Achaeta scute complex, and in vertebrates genes of the neurogenin group
Freed by the initial suppression by its neighbour, the neuroblast can fully activate proneural genes such as neurogenin (Fig. 16.10), whereas the neighbours switch on other genes. In prospective epidermal cells of Drosophila the epidermis-specific Enhancer of split complex, Esp-Cl, acts as an opponent of the neurogenic Achaete scute complex, As-C ; in the mouse HES fulfils the function of the opponent.
Later in the segregation of the future neurons from glial cells the Notch/Delta system commences activity again. The winner in this renewed duel will become the neuron, the loser will become the glial cell.
16.2.5 The Primary Neuroblasts Emerge on the Neural Plate in Six Stripes Which Express Different Homeobox Genes and Display an Intriguing Similarity to the CNS in Insects
Even though initially proneural genes are switched on in the entire neural plate this does not mean that all cells are immediately committed to become neuroblasts. When neuron-specific genes were detected and their expression pattern was visualized by in-situ hybridization unexpected patterns were found: On the neural plate three rows each of primary neurons on both sides of the midline became visible (Fig. 16.11). The two rows adjacent to the midline contain future motor neurons, the next rows contain future interneurons (plus glia), while the lateral rows are displaced outside the closing neural tube and become the neurogenic cells of the neural crest; these displaced neurogenic cells migrate from the margin of the tube to form the spinal ganglia (dorsal root ganglia) or provide the peripheral glia (see Figs. 16.17 and 16.18).
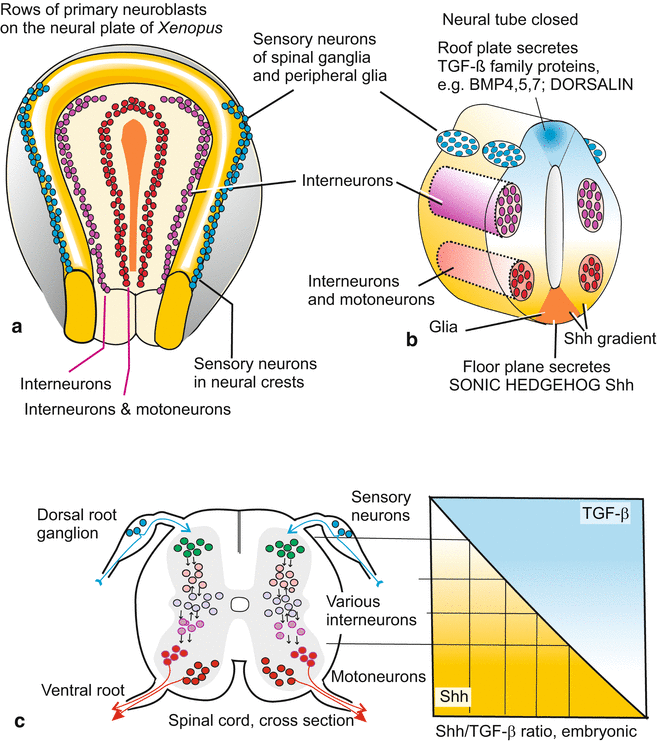
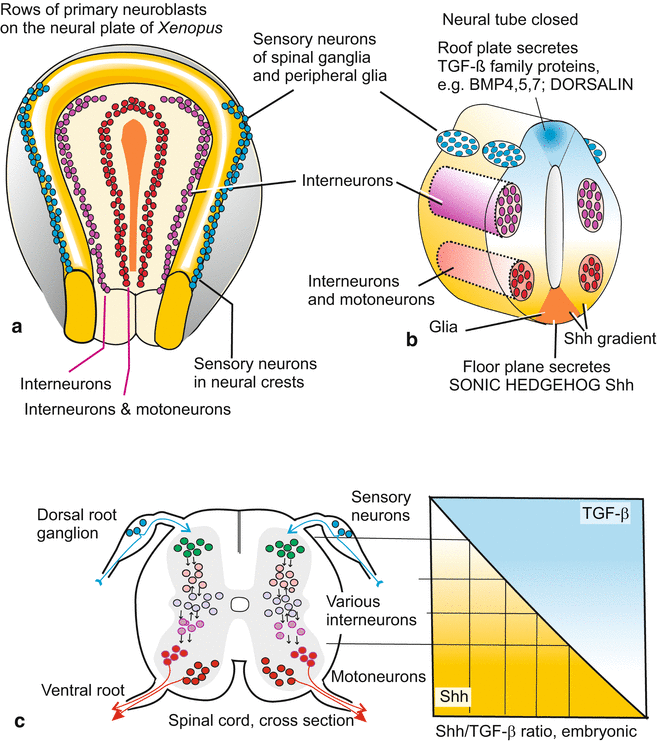
Fig. 16.11
(a–c) Fates of neuroblasts along the dorso-ventral axis of the developing neural tube, and factors involved in decision making. (a) Rows of primary neuroblasts on the neural plate of a frog neurula. (b) Neural tube arisen from the neural plate. (c) The SHH gradient and the complementary gradient of TGF-β molecules act as antagonistic morphogens. Their local ratio provides for selective and regio-specific activation of transcription factors which in turn define distinct neuronal fates
The genome of Drosophila encompasses, not very surprisingly, neuroblast-specific genes homologous to those of the vertebrates; they are expressed – and this was completely unexpected – in six longitudinal stripes as well (Fig. 16.12), the strongest argument in favour of a basic homology of these central nervous systems which are so widely different in their terminal structure. Even when it comes to subdivide the nervous system into brain and spinal cord, or brain and ventral cord, respectively, and to further regionalize the CNS along the longitudinal body axis of the embryo one sees homologous genes expressed in similar patterns, for example genes of Hom/Hox B group (Fig. 16.13).
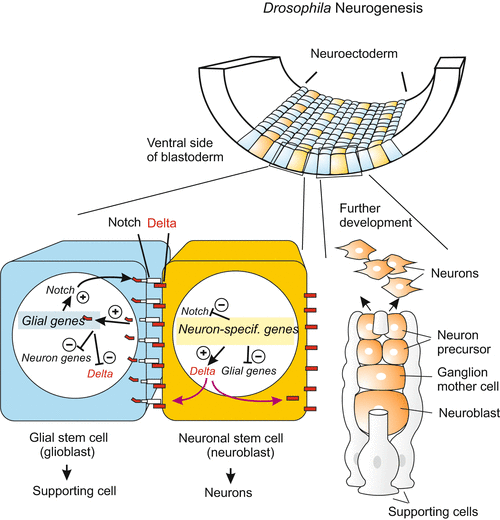
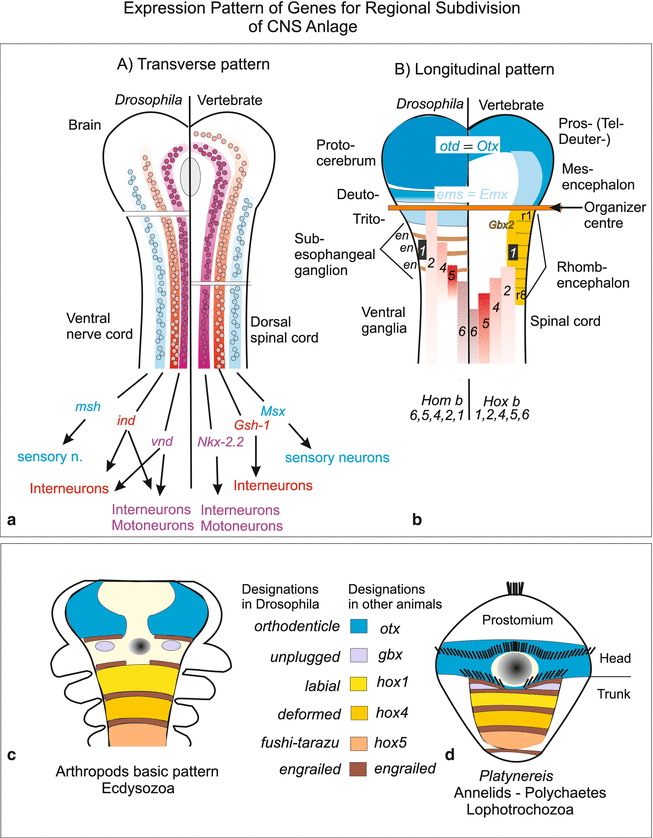
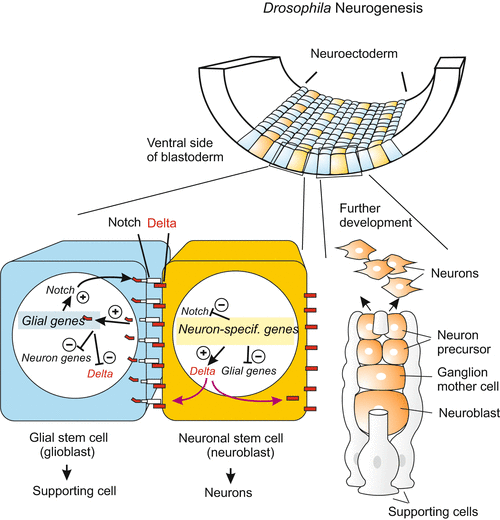
Fig. 16.12
Neurogenesis in insects. Also in the separation of the primary neuroblasts from the glia-type supporting cells the NOTCH/DELTA system is called into action
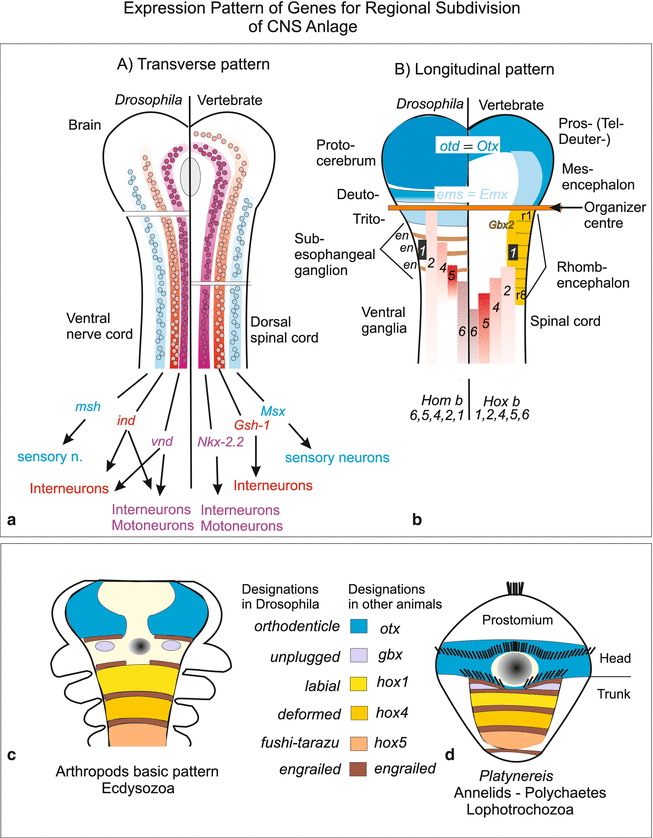
Fig. 16.13
Expression pattern of homeobox genes which contribute to the large-scale subdivision of the central nervous system CNS. (a, b) Expression pattern of homeobox genes in the anlage of the CNS in Drosophila and in vertebrates in comparison. (a) Genes programming the first rows of neuroblasts are expressed in lateral stripes. Genes marked with identical colour in Drosophila and vertebrates have similar nucleotide sequences and therefore are considered to be homologous. (b) Expression pattern of homologous Hox genes along the longitudinal body axis of the future CNS. In the embryo of Drosophila a series of genes (here otd, ems, en) is expressed in transverse stripes whereas the homologous genes in vertebrates are expressed in longitudinal stripes (Otd/Otx = orthodenticle, ems/Emx = empty spiracles, en = engrailed). The Hox genes (=Hom genes) of Drosophila are designated with different names on historical grounds: 1 = lab, 2 = pb, 4 = Dfd, 5 = Scr; 6 = Antp (see also Fig. 4.39). (c, d) Comparison of the expression and names of homeobox genes in arthropods and the polychaete Platynereis. (a) after Arendt and Nübler-Jung (1999); (b) after Cohen and Jürgens (1990) and Reichert (2005); (c, d) after Steinmetz et al. (2011)
16.3 The Growing CNS and Stem Cells
16.3.1 The Growing CNS: New, Secondary Neuroblasts Are Generated by Asymmetric Division of Stem Cells at a Rapid Speed
When in the growing human embryo the neural tube gives rise to the enlarging brain and to the extending spinal cord the existing neuroblasts must be increased in number. In embryonic development a human brain grows at an average speed of about 250,000 new nerve cells per minute up to several billions of nerve cells. In addition a tenfold higher number of supporting glial cells must be generated. Also subsequent to the embryonic phase and into postnatal life the brain grows in volume and mass by newly generated nerve and glial cells until by the end of puberty the maximum size of approximately 1011 nerve cells and 1012 supporting glial cells (microglia = immune cells) is reached.
The proliferation of neuronal cells (neurons and glia cells) is brought about by an epithelium-like layer of neurogenic stem cells (progenitor cells) called neuroepthelium or ependyma which lines the lumen of the canal of the spinal cord and the ventricles of the brain where supply of nutrients is best. This neuroepithelium remained as a two-dimensional layer when the six stripes of primary neuroblasts were segregated out. It contains stem cells which give rise to further populations of neuronal and glial progenitors.
The processes of the secondary neurogenesis can, of course, not be investigated in humans. The avian embryo, and currently transgenic, transparent fishes, and with substantial restrictions the mouse embryo, must act as model and proxy. In the zebrafish Danio novel techniques enabled time-lapse monitoring of living transgenic individuals expressing GFP-coupled fluorescing proteins in the stem cells and their derivatives. The recordings partially corroborated, but partially corrected, findings on histological preparations of mouse embryos. The processes are strange. In the course of each mitotic cycle the nuclei in the elongated stem cells migrate from a position close to the luminal surface to a position close to the peripheral surface where they undergo the S-phase of the cell cycle. Subsequently the nuclei return to the original position where mitotic division of the cell takes place (Fig. 16.14). The mitosis can be symmetric or asymmetric.
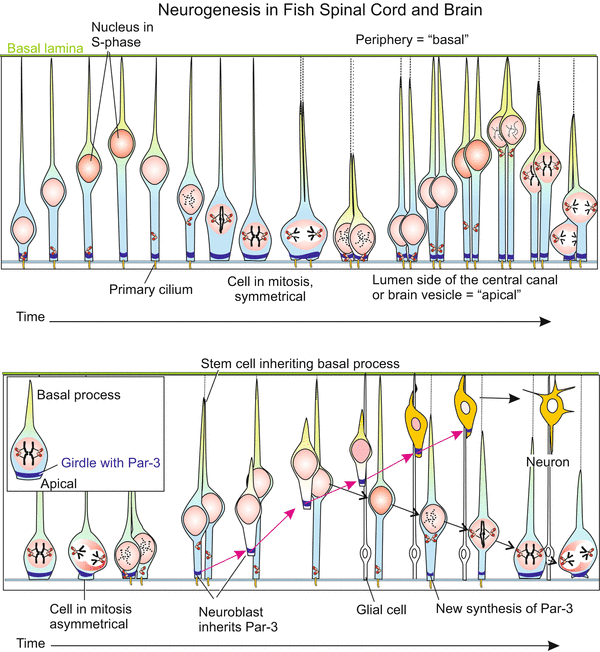
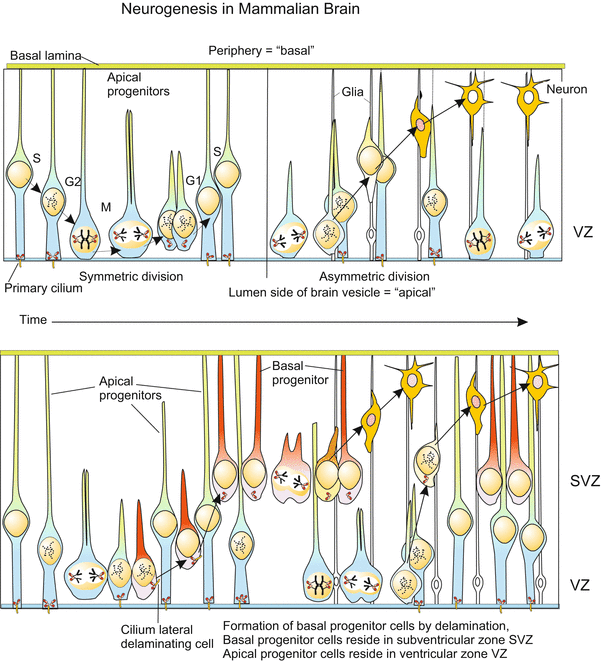
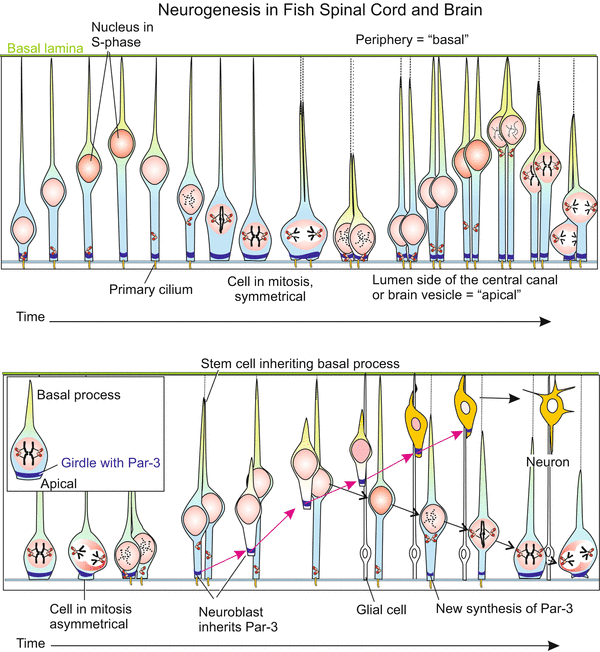
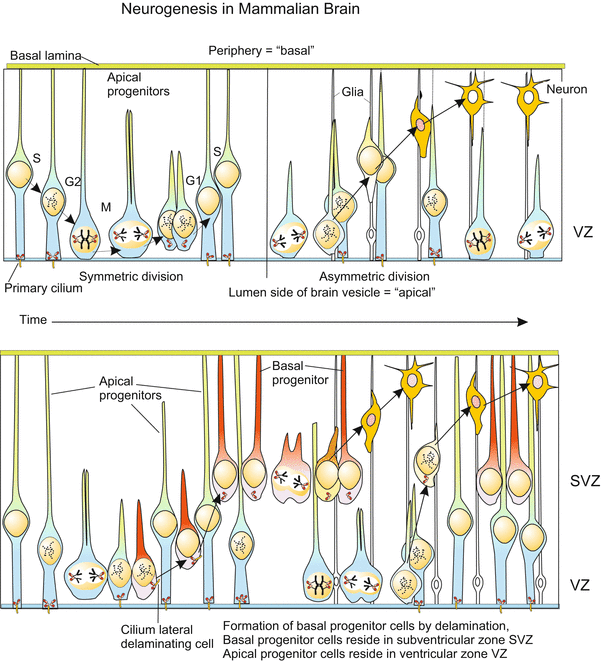
Fig. 16.14
Neurogenesis in the post-embryonic (postnatal) CNS. Enlargement is brought about by stem cells of the spinal cord located around the central channel- The mechanism has been analysed in transgenic zebrafishes by means of video recording of GFP-labelled specimens. Growth of the vertebrate brain through neurogenesis from stem cells of the ependyma, shown at the transition of the myelencephalon to the spinal cord in the fish Danio. In GFP transgenic fish the transparency of the body allows the tracking of stem cells labelled with GFP (Box 12.2) in vivo. By continuous video recording the migration of the cell nuclei in dividing stem cells was documented. The ependyma containing stem cells borders on the central, fluid-filled canal (in the brain on the ventricle). The side adjacent to the canal is designated “apical”. Shown is the production of further stem cells by symmetric division, and of secondary neuroblasts by asymmetric division. In asymmetric division the marker Par3-GFP is allocated to the future neuroblast. The nucleus of the second daughter cell returns to the apical home position and the cell resumes synthesis of Par2-GFP. After Alexandre 2010 (and talk 2011), supplemented after Taverna and Huttner 2010 (how far the basal processes exactly stretch in basal direction is not learned from these publications)
In symmetric mitoses certain cell components, for instance the protein Numb and the protein PAR-3 and a protein kinase aPKC, are uniformly distributed among the two daughter cells. As a result both daughter cells remain stem cells. Numb’s primary function in cell differentiation is as an inhibitor of Notch signalling, and suppressing Notch signalling is essential for maintaining self-renewal potential in stem and progenitor cells.
In the asymmetric mitoses these components are unequally distributed. One daughter cell retains Numb and remains stem cell. The daughter cell which inherits PAR-3 (but not Numb) becomes a postmitotic neuroblast. Postmitotic means that this cell will not divide anymore but will undergo terminal differentiation to become a neuron. But before this, the cell must leave its birthplace at the baseline, emigrate into peripheral layers and settle there (Figs. 16.14 and 16.15). The nucleus of the second cell which did not receive Par-3 but Numb, returns to the baseline and the cell re-synthesizes PAR-3, thus returning to its originals state as neurogenic stem cell.
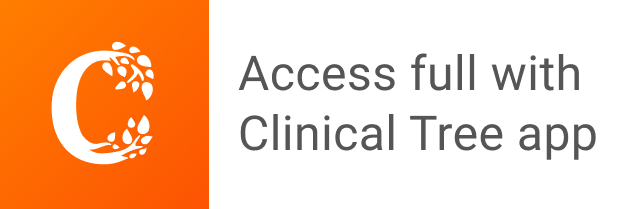