34 Mark G. Papich and Jim E. Riviere The tetracycline antibiotics were initially discovered in 1944, with the first one being chlortetracycline. These were isolated from the Streptomyces species (S. rimosus and S. aureofaciens), and later expanded to include the various semisynthetic products that include tetracycline, doxycycline, and minocycline. Oxytetracycline was discovered in 1948, tetracycline in 1953, doxycycline in 1967, and minocycline in 1972. The newest development is the glycylcyclines, which are derivatives of minocycline. Tigecycline is the only available representative of this group, which possesses better antimicrobial activity than older drugs (Agwuh and MacGowan, 2006). Tigecycline use in veterinary medicine has not been reported. The tetracyclines are a group of four-ringed amphoteric compounds (Figure 34.1) that differ by specific chemical substitutions at different points on the rings. As a group, the tetracyclines are acidic, hygroscopic compounds in aqueous solutions and easily form salts with acids and bases, with which they are commonly formulated. The most common salt form is the hydrochloride formulation, as is the case with oxytetracycline. However, some tetracyclines, especially oxytetracycline, are formulated with vehicles (excipients) to prolong absorption from the injection site. Some of the chemical and physical properties of the tetracyclines used in veterinary medicine today are listed in Table 34.1 . Table 34.1 Chemical and physical properties of tetracyclines Figure 34.1 Tetracyclines and other tetracycline structures. Tetracyclines have activity against both gram-positive and gram-negative bacteria, but resistance occurs frequently. They also have activity against atypical pathogens such as Mycoplasma, blood-borne pathogens (hemoplasma), and organisms such as Rickettsia transmitted by ticks and other parasites. Clinically accepted indications include abscesses, enteritis, Leptospirosis, pneumonia, bovine and swine respiratory disease, pododermatitis, treatment of tick-borne pathogens, skin and soft tissue infections, canine heartworm disease, and uterine infections. Many formulations have been administered in medicated water and for feed for production purposes (growth promotion). The US Food and Drug Administration (FDA) announced that as of 2017 the production uses of these antibiotics will be voluntarily withdrawn from livestock use. The tetracyclines comprise the largest group of antibiotics affected by this FDA order. The FDA believes that production use indications such as “increased rate of weight gain” or “improved feed efficiency” are no longer appropriate for the approved conditions of use for medically important antimicrobial drugs. These regulatory changes are provided in the Guidance for Industry (GFI) documents #209 and #213, which may be obtained from the FDA. These medications ordinarily added to feed and water of livestock will not be considered in this chapter because of their future status, and the levels administered are considered subtherapeutic. In addition, the pharmacokinetics of these formulations in the target species is incomplete. Tetracyclines possess antimicrobial activity by binding to the 30S ribosomal subunit of susceptible organisms. After binding to the ribosome, the tetracyclines interfere with the binding of aminoacyl-tRNA to the messenger RNA molecule/ribosome complex, thereby interfering with bacterial protein synthesis in growing or multiplying organisms (Gale and Folkes, 1953; Suzuka et al., 1966). Tetracyclines require an energy-dependent process to enter bacteria. One of the reasons for their selectivity against microorganisms is that mammalian cells lack this transport mechanism. Tetracyclines also have less affinity for mammalian ribosomes than bacterial ribosomes. Because the binding to the ribosome target is a reversible process, the drug concentrations must be maintained throughout the dose interval and these drugs are generally considered bacteriostatic. Details are described in Section Pharmacokinetic–Pharmacodynamic Properties. Because the tetracyclines have been used over many years in veterinary and human medicine, resistance is common and occurs across all groups of bacteria. The mechanisms of acquired resistance include: (i) energy-dependent efflux of antibiotic (membrane efflux proteins), or (ii) altered target whereby the ribosome is protected from binding of tetracyclines (Chopra et al., 1992). A third mechanism whereby the drug is attacked by enzymes liberated by the bacteria is possible. The genes mediating resistance may be carried on plasmids or transposons. Resistance to one tetracycline will generally produce cross-resistance to the others in the group. One of the exceptions is for Staphylococcus spp., which is discussed in Section Antimicrobial Spectrum and Clinical Uses. The newest tetracycline, tigecycline, is active against many organisms (e.g., methicillin-resistant staphylococci) that are resistant to older tetracyclines (Agwuh and MacGowan, 2006). For susceptibility testing, tetracycline may be used to test all others in the class (CLSI, 2013; CLSI, 2015). Specific breakpoints are also available for minocycline and doxycycline in dogs, and doxycycline in horses (Table 34.2 ). For veterinary isolates, the susceptible breakpoint varies among species and is lower than the human breakpoints, which should be considered when interpreting susceptibility tests. The breakpoint for susceptible organisms in humans is ≤4 μg/ml for all organisms, except streptococci, which is ≤2 μg/ml. Table 34.2 Tetracycline breakpoints for susceptibility testing. Source: CLSI, 2015. S, susceptible; I, intermediate; R, resistant. aThe results of tetracycline susceptibility tests are used to predict susceptibility for chlortetracycline and oxytetracycline. Isolates susceptible to tetracycline are susceptible to minocycline and doxycycline. Based on an evaluation of tetracycline pharmacokinetic–pharmacodynamic properties (PK-PD), the effectiveness is best expressed as a ratio of the area-under-the-curve for a 24-hour interval to the minimum inhibitory concentration (AUC24/MIC) (Agwuh and MacGowan, 2006). The optimal AUC/MIC ratio is in the range of 25–40, with Andes and Craig (2007) suggesting a value of 25. In veterinary studies this has been explored insufficiently. One study (Prats et al., 2005) examined PK-PD parameters after administration of doxycycline to swine in drinking water at 10 mg/kg. They reported high AUC/MIC ratios ranging from 60 for Pasteurella, 155 for Mycoplasma, and 585 for Bordetella. For Actinobacillus, which exhibits a higher MIC, the AUC/MIC was only 13. However, these ratios did not factor the protein binding, which is at least 90% (Table 34.3 ). Using a fraction unbound (fu) value of 0.1, reduces these ratios substantially. Ideal AUC/MIC ratios from their study would be achieved only for Bordetella bronchiseptica. Table 34.3 Protein binding of tetracyclines in various species (references provided in text) For Staphylococcus isolates from dogs, substantial PK-PD analysis was performed for doxycycline and minocycline (Maaland et al., 2013, 2014; Hnot et al., 2015a). It was concluded that AUC/MIC was the parameter that should be used for analysis, and a AUC/MIC ratio of 25 of the unbound fraction was optimum for predicting susceptibility and deriving dosages administered. Tetracyclines can be administered intravenously (most tetracyclines) or intramuscularly (oxytetracycline). The oral route also has been used (Table 34.4 ). Although some formulations (e.g., chlortetracycline and tetracycline) have been administered in feed and water for pigs, cattle, and poultry, the systemic effects of this route of administration may be much less than anticipated. In a study in which oxytetracycline was fed to pigs (Hall et al., 1989) the authors concluded that feeding this medication at a rate of 0.55 g/kg of feed resulted in plasma concentrations so low that only highly susceptible bacteria would be inhibited, with plasma concentrations reaching only one-tenth of the MIC for most pathogens. Several studies have examined use of tetracyclines added in subtherapeutic concentrations to feed rations (in particular, chlortetracycline) (Zinn, 1993; Jones et al., 1983; Dawson et al., 1983; Williams et al., 1978; Quarles et al., 1977; Richey et al., 1977; Nivas et al., 1976). The explanation for low oral absorption is not clear, but may be multifactorial. Tetracyclines are zwitterions and ionized at physiological pH values (see Table 35.1). Although the tetracyclines are relatively lipophilic drugs, they are ionized in the gastrointestinal tract and may not cross membranes easily. The oral absorption of tetracycline can be reduced in the presence of food (Nielsen and Gyrd-Hansen, 1996; Hnot et al., 2015b). Tetracyclines can easily chelate to polyvalent cations, which decreases the absorption several-fold. Thus, tetracycline oral absorption can be decreased with the coadministration of food, dairy products, polyvalent cations (i.e., Ca2+, Mg2+, Fe2+, Al3+), kaolin/pectin preparations, iron-containing supplements, and antacids (Weinberg, 1957; Waisbren and Hueckel, 1950; Harcourt and Hamburger, 1957; Neuvonen et al., 1970; Hägermark and Hoglund, 1974; Gothoni et al., 1972; KuKanich et al., 2014; KuKanich and KuKanich, 2015). When doxycycline or minocycline were administered with sucralfate (containing aluminum) oral absorption was significantly reduced unless the sucralfate was administered 2 hours after the tetracycline (KuKanich et al., 2014; KuKanich and KuKanich, 2015). Table 34.4 Comparison of oral absorption of tetracyclines in animals (mean values of studies reported; references listed in text) Once absorbed, tetracyclines bind to plasma proteins to varying degrees in each species with doxycycline being greater than 80% in most animals (Riond and Riviere, 1989b) (Table 34.3 ). The high protein binding for some drugs may limit the microbiologically active fraction in the plasma. For the agents that are not restricted by protein binding, tetracyclines are widely distributed throughout most tissues of the body, including intracellular sites. Tables 34.5 –34.9 show the pharmacokinetic variables for some of these drugs. High protein binding can limit tissue distribution. Bidgood and Papich (2003) showed that plasma protein binding for doxycycline was high (91.75%), which drastically limited the distribution into the tissue fluid. The same property was shown with doxycycline in horses (Davis et al., 2006). The plasma protein binding of 82% in horses reduced distribution to tissue fluid. Maaland et al. (2014) showed that plasma protein binding of minocycline limited the distribution to approximately 50% of the dose administered. Protein binding has less of an effect on distribution to intracellular sites and joint fluid (see below in this section). Table 34.5 Pharmacokinetic parameters of chlortetracycline in some food-animal species NR, information not reported; IV, intravenous; MRT, mean residence time. Table 34.6 Pharmacokinetic parameters of tetracycline in some species NR, information not reported; IV, intravenous; IA, intraarterial; MRT, mean residence time. Table 34.7 Pharmacokinetic parameters of oxytetracycline in some species NR, or blank space, information not reported; IV, intravenous; PO, per os; MRT, mean residence time. All formulations were reported to be or are assumed to be HCl unless otherwise noted. Vd, apparent volume of distribution (Vd/F for non-IV dose forms); T1/2, elimination half-life, or terminal half-life (for multicompartment models); Clearance, systemic clearance (CL/F for non-IV dose forms). aOxytetracycline dihydrate formulation tested. Table 34.8 Some pharamcokinetic parameters of doxycycline in some species CRI, constant rate infusion; IV, intravenous; NR, information not reported, Cmax, peak concentration; Vd, apparent volume of distribution (Vd/F for oral dose); T1/2, half-life (terminal half-life for multicompartment models). Clearance, systemic clearance (CL/F) for non-IV dose forms. All values listed are means from the study. Blank cells indicates that information was not available.
Tetracycline Antibiotics
General Pharmacology of Tetracyclines
Drug
Molecular weight
pKa
Chlortetracycline
478.88
3.3, 7.4, 9.3
Doxycycline
462.46
7.75
Minocycline
457.48
8.25
Oxytetracycline
460.44
7.75
Tetracycline
444.43
8.3, 10.2
Mechanism of Action
Resistance:
Susceptibility testing:
Drug
Bacteria
Animal
S (μg/ml)
I (μg/ml)
R (μg/ml)
Tetracyclinea
Mannheimia haemolytica
Cattle
≤ 2
4
≥ 8
Pasteurella multocida
Histophilus somni
Tetracyclinea
Actinobacillus pleuropneumoniae
Pigs
≤ 0.5
1
≥ 2
Pasteurella multocida
Streptococcus suis
Tetracycline
Staphylococcus pseudintermedius
Dog
≤ 0.25
0.5
≥ 1
Doxycycline
Staphylococcus pseudintermedius
Dog
≤ 0.12
0.25
≥ 0.5
Doxycycline
Staphylococcus aureus, Streptococcus equi ssp. zooepidemicus, Streptococcus equi ssp. equi, Escherichia coli
Horse
≤ 0.12
0.25
≥ 0.5
Minocycline
Staphylococcus pseudintermedius
Dog
≤ 0.5
1
≥ 2
Tetracycline
Enterobacteriaceae
Human
≤ 4
8
≥ 16
Tetracycline
Staphylococcus, Streptococcus
Human
≤ 2
4
≥ 8
Pharmacokinetic–Pharmacodynamic Properties
Drug
Species
% Protein binding
Chlortetracycline
Cows
47–51
Sheep
46–50
Doxycycline
Calves
92
Cats
98
Horses
82
Dogs
91–92
Pigs
93
Sheep
84–90
Turkeys
70–85
Oxytetracycline
Buffalo
42
Cows
18–22
Horses
50
Pigs
75.5
Sheep
21–25
Trout
55
Tetracycline
Cows
31–41
Sheep
28–32
Minocycline
Dog
65.8
Sheep
80
Absorption
Drug
Species
Systemic absorption (F%)
Chlortetracycline
Chickens
1
Turkeys
6
Pigs
6, 11, 19 (depending on the study and fasting conditions)
Oxytetracycline
Pigs
3–5
Fish
6
Turkeys
9–48
Tetracycline
Pigs
5, 8, 18, 23 (depending on the study and fasting conditions)
Dogs
40
Cats
50
Doxycycline
Pigs
21.2
Chickens
41.3
Turkeys
25, 37, 41, 63.5 (depending on age)
Horses
17.3 (intragastric); 6 as a top dressing; higher in foals
Dogs
53 (hyclate), 33.5 (monohydrate)
Dogs
61.85 (summary of 4 studies)
Calves
70
Minocycline
Dogs
50.3
Cats
62
Distribution
Dose
Vd
t1/2
Clearance
Species
(mg/kg)
Route
(l/kg)
(hour)
(ml/min/kg)
Reference
Turkey
0.9
IV
0.2284
0.877
3.77
Dyer, 1989
Pigs
11.0
IV
1.3883
NR
0.3071
Kilroy et al., 1990
Pigs
10
IV
0.7
3.6 (MRT)
3.33
Nielsen and Gyrd-Hansen, 1996
Pigs
39.9
Oral
—
8.7 (MRT)
—
Nielsen and Gyrd-Hansen, 1996
Calves (milk fed)
11.0
IV
3.34
8.89
260.52 l/h/kg
Bradley et al., 1982
Calves (conventionally fed)
11.0
IV
1.93
8.25
162.12 l/h/kg
Bradley et al., 1982
Dose
Vd
t1/2
Clearance
Species
(mg/kg)
Route
(l/kg)
(hour)
(ml/min/kg)
Reference
Gilts
11
IA
1.06
NR
0.4
Kniffen et al., 1989
Chickens
65
IV
0.174
2.772
1.632
Anadon et al., 1985
Rabbits (male and female)
10
IV
1.047
2
6.1
Percy and Black, 1988
Channel catfish (Ictalurus punctatus) (27°C)
4
IV
0.513
16.5
0.365
Plakas et al., 1988
Pigs
11
IV
4.5
16
3.1
Kniffen et al., 1989
Pigs
9.6
IV
1.2
5.6 (MRT)
3.5
Nielsen and Gyrd-Hansen, 1996
Pigs
46.4
Oral
—
9.0 (MRT)
—
Nielsen and Gyrd-Hansen, 1996
Dose
Vd
T1/2
Clearance
Species
(mg/kg)
Route
(l/kg)
(hour)
(ml/min/kg)
Reference
Horses
10
IV
0.6728
12.953
0.6583
Horspool and McKellar, 1990
Ponies
10
IV
1.0482
14.949
1.013
Horspool and McKellar, 1990
Donkeys
10
IV
0.7765
6.464
1.523
Horspool and McKellar, 1990
Horses (adult)
2.5
IV
1.35
10.5
NR
Pilloud, 1973
Pigs
10
IV
1.49
5.99
2.88
Pijpers et al., 1991
Pigs (normal)
50
PO
1.44
5.92
Pijpers et al., 1991
Pigs (pneumonia)
50
PO
1.9
14.1
Pijpers et al., 1991
Pigs
20
IV
5.18
3.68
4.15
Mevius et al., 1986b
Cows (adult)
2.5
IV
1.04
9.12
NR
Pilloud, 1973
Dairy cows
5
IV
0.917
2.63
1.24
Nouws et al., 1985a, 1985b
Dairy cowsa
5.23
IV
1.01
2.58
1.45
Nouws et al., 1985a, 1985b
Veal calves
40
IV
18.144
7.34
2.246
Meijer et al., 1993a
Veal calves
20
IV
18.541
2.167
Meijer et al., 1993a
Calves (3 weeks old)
7.54
IV
2.48
13.5
Nouws et al., 1983
Calves (12 weeks old)
6.88
IV
1.52
8.8
Nouws et al., 1983
Calves (14 weeks old)
17
IV
1.83
10.8
Nouws et al., 1983
Buffalo calves (female)
22
IV
0.32
3.6
1.02
Varma and Paul, 1983
Dogs
5
IV
2.096
6.02
4.23
Baggot et al., 1977
Rabbits
10
IV
0.668
1.32
14.6
McElroy et al., 1987
Turkeys
1
IV
3.622
0.7298
3.6579
Dyer, 1989
Rainbow trout
5
IV
2.988
81.5
0.423
Black et al., 1991
African catfish
60
IV
1.33
80.3
0.19
Grondel et al., 1989
Red-necked wallaby
40
IV
2.041
11.4
NR
Kirkwood et al., 1988
Foal
59
IV
1.95–2.2
6.7–7.3
3.3
Papich et al., 1995
Cattle
20
IM
3.34
21.6
—
Craigmill et al., 2004 (meta-analysis)
Cattle (young)
20
IV
0.94
5.67
—
Toutain and Raynaud, 1983
Calves
40
IM
—
23.9
—
TerHune and Upson, 1989
Calves (healthy)
11
IV
2.32
11.8
3.35
Ames et al., 1983
Calves (disease)
11
IV
3.6
14.8
4.01
Ames et al., 1983
Pigs
9.5
IV
1.4
6.5 (MRT)
3.67
Nielsen and Gyrd-Hansen, 1996
Pigs
45.5
Oral
—
10.3 (MRT)
—
Nielsen and Gyrd-Hansen, 1996
Sea turtles
25
IV
18.4
66.1
4.8
Harms et al., 2004
Sea turtles
25
IM
28.5 (Vd/F)
61.9
5.3 (CL/F)
Harms et al., 2004
Alligator
10
IV
0.77
74.1
0.12
Helmick et al., 2004
Cmax
T1/2
Clearance
Species
Dose (mg/kg)
Route
(μg/ml)
Vd (l/kg)
(hour)
(ml/min/kg)
Reference
Pigs (9 weeks old)
20
IV
0.53
4.04
1.67
Riond and Riviere, 1989
Pigs
12
Oral
—
7.2
—
Prats et al., 2005
Pigs
10.5
IV
0.89
4.2
2.8
Baert et al., 2000
Pigs
10.5
Oral
0.97
2.9
2.9
Baert et al., 2000
Horses
20
Oral
0.91
—
11.8
—
Davis et al., 2006
Horses
10
Oral
2.54
–
8.5
–
Womble et al., 2007
Horses
20
Oral
2.89
–
11.9
–
Womble et al., 2007
Horses
10
Oral
0.48
–
13.8
–
Winther et al., 2011
Horses
5
Oral
0.37
–
15.08
–
Schnabel et al., 2010
Calves
5
IV
–
9.5
1.2 (mg/l)
Meijer et al., 1993b
Calves (functional rumen)
20
IV
1.31
14.9
1.07
Riond et al., 1989
Calves (nonfunctional rumen)
20
IV
1.81
9.9
2.2
Riond et al., 1989
Cats
5
IV
0.34
4.56
1.09
Riond et al., 1990
Dogs
5
IV
0.93
6.99
1.72
Riond et al., 1990
Dogs
5
IV
1.468
10.36
1.68
Wilson et al., 1988
Dogs
0.1 CRI mg/
IV
0.65
4.56
1.66
Bidgood and Papich, 2003
kg/h
Dogs
5–10
Oral
4.53
1.67
12.6
1.68
(summary of 3 studies)
Goats (lactating)
5
IV
9.78
16.63
6.91
Jha et al., 1989
Stay updated, free articles. Join our Telegram channel
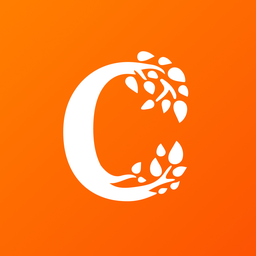
Full access? Get Clinical Tree
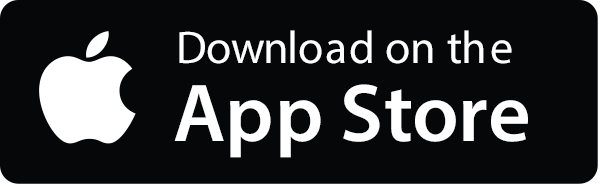
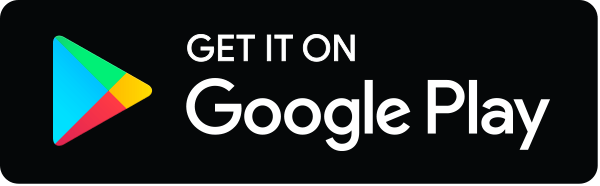