Douglas C. Hodgins Raveendra R. Kulkarni and Patricia E. Shewen The wide variety of bacterial pathogens that successfully infect and continue to affect humans and animals, despite the robust capabilities of host immune systems, demonstrates that these pathogens can evade, block, defeat, inactivate, overcome, or otherwise subvert immune defenses. With recent advances in experimental methods and analysis, understanding of the complexities of the immune system has progressed relentlessly. Insights into the molecular details of gene regulation, post‐transcriptional modifications, cellular signaling pathways, apoptosis, autophagy, and other cellular processes are accumulating rapidly. Investigations into mechanisms used by bacterial pathogens to subvert these processes lag somewhat behind, but it is clear that bacterial pathogens can counteract host defenses at the same high, or perhaps higher, level of sophistication. Indeed, some bacterial effectors are versatile in that they can target multiple points of host susceptibility. While the initial evidence for subversion of immune defenses may originate from computational analysis of extensive databases, confirmatory evidence comes from in vitro studies using established cell lines and model systems. However, in vitro studies have their own limitations in ascertaining whether proposed mechanisms actually enhance bacterial replication, persistence, or transmission in vivo in target host species. Some bacteria have multiple, overlapping mechanisms that must be deployed, in sequence, at critical time points to be advantageous to the pathogen. The material summarized in this chapter is presented in the context of existing evidence, with the recognition that future work may show that some of the mechanisms do not have clinical relevance. Although the subject of this book is bacterial pathogens of animals, some pathogens of humans have been included to illustrate certain precise mechanisms of subversion that have been well studied in the context of human medicine. Animal pathogens doubtless use some of these mechanisms, but this awaits confirmation. Immune defenses have conventionally been classified as innate or adaptive. Innate defenses consist of cells and secreted substances that are not specific for particular pathogens. Classically, innate responses have been considered to lack memory. It was thought that second exposure of innate cells to a pathogen would lead to responses similar in magnitude to those following primary exposure. It is now evident that primary infection with some pathogens will sensitize innate cells such that secondary responses, to the same pathogen (or indeed an unrelated pathogen), will be of larger magnitude. This characteristic of innate defenses has been termed “trained immunity” (Netea et al. 2020). Innate defenses include epithelial barriers to prevent bacterial penetration, clearance of mucous and trapped bacteria from the trachea by the action of ciliated epithelial cells, antimicrobial peptides, complement components, natural killer cells, and phagocytic cells such as neutrophils, macrophages and dendritic cells. Adaptive defenses include immune responses that are clonally defined, including antibodies generated by antigen‐specific B lymphocytes, and T lymphocytes specific for fragments of bacterial components. Adaptive responses require time to develop but typically are associated with immune memory; re‐exposure to the same pathogen leads to responses of greater magnitude, with accelerated kinetics. Bacteria of different species, serotypes, and strains have diverse mechanisms to subvert innate and adaptive immune systems at multiple points of defense and may intervene at multiple points simultaneously. Antimicrobial peptides comprise a large family of peptides with bactericidal activity against Gram‐positive and/or Gram‐negative organisms (Guerra et al. 2017). They are produced by neutrophils and macrophages, by epithelial cells of the skin, respiratory, genitourinary, and gastrointestinal tracts. Beta‐defensins are expressed by epithelial cells of the trachea following binding of lipopolysaccharides (LPS) to Toll‐like receptor 4 (TLR4), with signaling via a nuclear factor kappa B (NF‐κB) pathway. In vitro and in vivo studies indicate that an unidentified virulence factor of Bordetella bronchiseptica, delivered by a type‐III secretion system (T3SS) can sequester NF‐κB in the cytoplasm of tracheal epithelial cells, reducing expression of β‐defensins (Ryan et al. 2018). Staphylococcus aureus expresses staphylokinase, which binds α‐defensins to form inactive complexes. In addition, multiple peptide resistance factor (MpdF) and DltB block binding of cationic antimicrobial peptides to S. aureus by generating a net positive charge on bacterial surfaces (Guerra et al. 2017). The lower respiratory tract is exposed continuously to microorganisms in inspired air. Some of these organisms become trapped in the mucous layer covering the epithelial cells of the larger airways and are transported upstream by the movement of cilia on the epithelial cells (mucociliary escalator). Bordetella pertussis secretes tracheal cytotoxin (TCT), which induces production of nitric oxide within tracheal epithelial cells, impairing their ability to clear mucus. TCT alone or in combination with LPS induces disruption of tight junctions and necrosis of epithelial cells (Kessie et al. 2021). Epithelial barriers constitute the first line of defense, and most species of pathogenic bacteria have mechanisms to breach these barriers. These mechanisms include adhesins binding host‐cell receptors or host‐cell extracellular matrix (e.g. Staphylococci), long polar fimbriae to enhance bacterial uptake by M cells of intestinal Peyer’s patches (e.g. Salmonella enterica serovar Typhimurium, Gonzales et al. 2017), T3SS that inject bacterial proteins into host cells to act as receptors (e.g. enteropathogenic Escherichia coli, EPEC). These mechanisms are described in detail in other chapters and are not discussed further here. Studies of pathogen‐epithelial cell interaction have revealed syndecan‐1 (Sdc1), a major cell‐surface heparan sulfate proteoglycan of epithelial cells, as a target for microbial adhesion (Aquino et al. 2018). Sdc1 knockout mice are significantly less susceptible to several bacterial infections compared with wild‐type mice, suggesting that Sdc1 is important for bacterial adhesion and cell entry. Pathogens that target Sdc1 include Klebsiella pneumoniae, Listeria monocytogenes, and S. aureus (Hayashida et al. 2015; García et al. 2016). The complement system consists of three overlapping enzyme pathways that contribute to innate immunity by enhancing phagocytosis of foreign material, generating proinflammatory molecules (anaphylatoxins), and killing pathogens by formation of membrane pores. The complement system also contributes to development of adaptive immunity (Carroll and Isenman 2012) by enhancing B cell activation and facilitating memory antibody responses. The classical, alternative and lectin complement pathways differ in their activation mechanisms but are identical in their terminal components. All three pathways generate C3‐convertase complexes bound to bacterial surfaces. These complexes cleave complement protein C3 to generate C3b fragments. Surface‐bound C3b, in association with the C3‐convertase, mediates cleavage of C5 to C5b, initiating assembly of a membrane‐spanning pore (C5b‐C6‐C7‐C8‐C9, the membrane attack complex, MAC). Insertion of sufficient of these pores in membranes may lead to death of the pathogen. Delves et al. (2017) can be consulted for mechanistic details. Bulky LPS and capsular polysaccharides create a barrier between surface‐bound C3b and bacterial membranes, which are the targets for MACs. Thus, Gram‐negative bacteria of smooth colony type (LPS with long O‐chains) are more resistant to complement‐mediated lysis than bacteria of rough colony type (LPS with incomplete O‐chains). Bacteria with thick polysaccharide capsules are more resistant than non‐encapsulated strains to complement attack. In general, Gram‐positive bacteria are resistant to lysis by MAC because of their thick peptidoglycan layers (Krukonis and Thomson 2020). C1q, the first component in the classical complement pathway, binds directly to bacterial cell wall components, such as lipoteichoic acid of Gram‐positive bacteria, or to C‐reactive protein bound to bacterial phosphocholine, or to immune complexes containing immunoglobulins (Ig) M or G. Protein A of S. aureus, binds the Fc region of IgG, preventing binding by C1q and blocking complement activation by the classical pathway. C1 esterase inhibitor (C1inh), a host regulatory protein, inhibits the serine protease activity of C1r and C1s (classical pathway) and mannan‐binding lectin serine protease 1 (MASP‐1) and MASP‐2 (lectin pathway), inhibiting formation of C3‐convertases. B. pertussis, a respiratory pathogen of humans, expresses virulence‐associated gene 8 (Vag8), which binds host C1inh to the bacterial surface, leading to resistance to serum‐mediated killing. B. bronchiseptica, a respiratory pathogen of dogs and pigs, expresses a similar Vag8 protein (99% amino acid identity), but it is uncertain whether it binds C1inh of the relevant host species (Marr et al. 2011). C4b‐binding protein (C4BP) is a host regulatory protein that degrades C4b preventing activation of the classical and lectin pathways. Fibrinogen‐binding proteins Fib2 and Fib3 of Streptococcus agalactiae bind C4BP in vitro, suggesting a role in degradation of C4b in vivo (Margarit et al. 2009). Sialic acids are common components of glycoconjugates on eukaryotic cells. Host complement regulatory factor H binds sialic acids and inactivates C3 convertase complexes to protect host cells. Some bacteria incorporate sialic acid into their capsules or LPS; others capture sialic acid released from host cells. S. aureus expresses SdrE and Borrelia burgdorferi expresses multiple complement regulator‐acquiring surface proteins (CRASPs) which bind host factor H, limiting C3 convertase activity (Kraiczy and Stevenson 2013). S. aureus also produces staphylokinase, which activates host plasminogen to degrade C3b and IgG, and staphylococcal complement inhibitor (SCIN), which inactivates the C3‐convertase of the alternative pathway. Byproducts of the complement cascades contribute to immune function. C3a, C4a, and C5a, small fragments generated by the cleavage of C3, C4, and C5, respectively, have proinflammatory effects. These anaphylatoxins are chemotactic for phagocytic cells and increase local vascular permeability facilitating delivery of soluble immune mediators. Chemotaxis inhibitory protein of S. aureus (CHIPS) binds the C5a receptor (C5aR) on neutrophils, reducing the inflammatory effects of C5a. S. pyogenes, produces a C5a peptidase which degrades C5a (Guerra et al. 2017). All three complement pathways generate reactive C3b which binds covalently to nearby microbial surfaces or antigens. Host proteins cleave C3b yielding fragment C3d, still covalently bound to its target. C3d acts as a molecular adjuvant, binding complement receptor 2 (CR2) on antigen‐specific B cells and follicular dendritic cells, enhancing primary and memory antibody responses. S. aureus secretes extracellular fibrinogen‐binding protein (Efb) and Staphylococcal binder of immunoglobulin (Sbi), which bind C3d with high affinity, blocking interaction with CR2 and suppressing antibody responses (Carroll and Isenman 2012). Microorganisms that penetrate into tissues are internalized by tissue‐resident macrophages, infiltrating blood monocytes and neutrophils. Various pattern‐recognition receptors (; e.g. TLRs, macrophage mannose receptors, scavenger receptors, and fMet‐Leu‐Phe, fMLP, receptors) on the phagocytes identify pathogen‐associated molecular patterns (PAMPs) of bacteria, leading to activation of signaling pathways and proinflammatory responses. Engulfed bacteria are enclosed within a phagosome vacuole, which then fuses with the lysosome to form a phagolysosome. As the pH inside this fused vacuole decreases, toxic reactive substances such as nitric oxide, superoxide anion, and hydrogen peroxide are released in an “oxidative burst” or “respiratory burst,” and, with the assistance of antimicrobial peptides, non‐pathogenic bacteria are killed. Pathogenic bacteria subvert this process in various ways (Figure 5.1; Table 5.1). B. bronchiseptica expresses an adenylate cyclase (AC) toxin‐hemolysin (Cya), which catalyzes production of cyclic adenosine monophosphate (cAMP) by phagocytic cells, inhibiting bactericidal oxidative burst responses (Hibrand‐Saint Oyant et al. 2005). Bacillus anthracis produces protective antigen, edema toxin, and lethal toxin, which work in concert to block expression of proinflammatory cytokines, impede motility of neutrophils, inhibit the oxidative burst in neutrophils and block apoptosis (Ehling‐Schultz et al. 2019). Streptococcus equi expresses an interleukin (IL)‐8 peptidase SpyCEP, which reduces the influx of neutrophils to sites of infection (Turner et al. 2009). Actinobacillus pleuropneumoniae, Moraxella bovis, uropathogenic E. coli and Mannheimia haemolytica secrete toxins of the repeats in the structural toxin (RTX) family. RTX toxins bind CD18 in leukocyte membranes, inducing pore formation and lysis of macrophages, neutrophils, and lymphocytes (Farias et al. 2015; Ristow and Welch 2019). Killing of phagocytes limits phagocytosis; release of inflammatory mediators by dying neutrophils may, however, have greater importance in disease pathogenesis. Figure 5.1 Microbial interference with phagocytosis and antigen processing. Broken arrows show pathway for microbial destruction. Numbers in boxes correspond to points vulnerable to microbial interference, as in Table 5.1. C′, complement; CR3/4, complement receptors; FcR, immunoglobulin Fc receptor; TLR, Toll‐like receptor. Table 5.1 Examples of bacterial subversion of uptake and killing. a) Numbers refer to boxes in Figure 5.1. Select bacterial species, including S. aureus and Pseudomonas aeruginosa, form biofilms consisting of structured communities of bacteria sheltered within extracellular matrices of extracellular DNA, polysaccharides and proteins. Bacteria within biofilms evade immune defenses by secretion of pore‐forming leukocidins and hemolysins, by limiting TLR2‐ and TLR9‐mediated signaling of macrophages thereby reducing expression of proinflammatory IL‐1β, and by limiting attachment of complement C3b, thus inhibiting phagocytosis (Schilcher and Horswill 2020). Numerous species of obligate or facultative intracellular pathogens escape from phagosomes into cytoplasm. Rickettsia use hemolysin C and phospholipase D to induce breakdown of phagosomal membranes. Following bacterial proliferation, actin polymerization is induced, forcing bacteria through host‐cell membranes into adjoining cells, forming membrane vacuoles in the process (Sahni et al. 2019). L. monocytogenes uses pore‐forming listeriolysin to escape phagosomes, and spreads from cell to cell in a similar fashion (Dowd et al. 2021). Research over the past five decades has documented mechanisms used by Mycobacterium tuberculosis to survive within macrophages. Secretion of protein tyrosine phosphatase (PtpA) and secreted acid phosphatase (SecM) blocks phagosomal acidification and fusion with lysosomes. For years, there was a near consensus that mycobacteria persist indefinitely in modified phagosomes without escaping into the cytosol. It is now evident that mycobacteria can escape via the ESAT‐6 secretion system‐1 (ESX‐1), type VII secretion system. Escape of mycobacteria into the cytosol triggers DNA sensing pathways, however, leading to expression of interferon (INF)‐β (Tiwari et al. 2019). Further research using in vivo models is needed to clarify mechanisms regulating the phagosome‐cytosol translocation. Chlamydiae are obligate intracellular bacteria that induce phagosomal modifications to persist and multiply within phagosomes. Chlamydiae in the infectious elementary body stage infect mucosal cells and differentiate into the replicating reticulate body stage within the phagosome. The phagosome is expanded and modified by the bacteria to form the inclusion vacuole, incorporating membrane resources of the endoplasmic reticulum and Golgi apparatus. As the bacteria replicate, the inclusion is expanded accordingly. Types 2SS and 3SS are used to deliver effectors to the lumen of the inclusion and to the inclusion membrane, respectively. Host cell transport pathways and nutrients are co‐opted to support chlamydial replication. Chlamydiae can downregulate expression of tumor necrosis factor (TNF) receptor‐associated factor‐3 (TRAF3) and inducible nitric oxide synthase (iNOS) and sequester NF‐κB to limit proinflammatory responses (Triboulet and Subtil 2019). M. tuberculosis survives within macrophage phagosomes by blocking acidification of the phagosomes. However, classically activated (M1) macrophages recover the ability to acidify phagosomes in response to IFN‐γ produced by pathogen‐specific T lymphocytes. M. tuberculosis in response can express a serine protease, Rv3671c, which restores and maintains a neutral intrabacterial pH (Biswas et al. 2010). Classically activated M1 macrophages can generate superoxide radicals, nitric oxide and peroxynitrite with the potential to kill phagocytosed M. tuberculosis. To maintain redox homeostasis M. tuberculosis uses low molecular weight thiols. In addition, secreted iron‐dependent superoxide dismutase A (SODA) and outer‐membrane‐associated copper‐dependent superoxide dismutase C (SODC) act as superoxide radical scavengers to limit oxidative stress. Alkyl‐hydroperoxide reductase‐C (AhpC) acts to reduce peroxynitrite (Piacenza et al. 2019). TLRs are a class of PRR that recognizes microbial products and signal through adapters myeloid differentiation primary response 88 (MyD88) and toll‐IL‐1 receptor‐domain‐containing adapter‐inducing IFN‐β (TRIF), to elicit NF‐κB‐dependent and mitogen‐activated protein kinase (MAPK)‐dependent transcription of proinflammatory cytokines. TRIF mediates a signaling cascade involving receptor‐interacting protein kinase 1 (RIPK1). Yersinia species effector protein YopJ facilitates acetylation of NF‐κB pathway mediators, preventing phosphorylation of RIPK1 at multiple sites, leading to reduced expression of proinflammatory cytokines, and induction of cell death (Philip et al. 2016). Successful bacterial pathogens evade innate responses mediated by TLR and nucleotide‐binding oligomerization domain (NOD) receptor signaling. Alteration of PAMP surfaces such as lipid A, flagella, and peptidoglycan has been studied extensively (Iwasaki and Medzhitov 2015). Some Gram‐negative pathogens, including Salmonella (Fernández et al. 2018), and Yersinia (Rosadini et al. 2015), can alter their lipid A acyl chains to evade recognition by TLR4. Leptospira LPS O‐antigens and associated lipoproteins resist TLR4‐mediated internalization by mouse macrophages. TRIF‐dependent nitric oxide responses and chemokine (C‐C motif) ligand 5 (CCL5) expression are suppressed, contributing to chronic leptospiral infections with persistent bacterial shedding (Bonhomme et al. 2020). Some intracellular bacterial pathogens prevent processing of peptidoglycan‐derived muropeptides and their detection by NOD1 and NOD2 cytosolic receptors (Ratet et al. 2017). Some bacterial pathogens interfere with signaling molecules upstream of NF‐κB nuclear translocation such as transforming growth factor‐β‐activated kinase 1 (TAK1), and immunoreceptor tyrosine‐based inhibitory motif (ITIM) molecules to hinder inflammatory responses. Yersinia pestis effector LcrV specifically targets the TLR2/6 pathway to divert a protective inflammatory response toward IL‐10 production. The Y. pestis effector YopJ blocks MAPK and TAK1 molecular signaling (Paquette et al. 2012), whereas Salmonella AvrA mediates intracellular survival by inhibiting MAPK4 and MAPK7 (Wu et al. 2012). Similarly, Helicobacter pylori and S. aureus produce effector proteins that can bind the ITIM‐bearing inhibitory receptor paired Ig‐like receptor B (PIR‐B) to reduce TLR‐induced inflammatory cytokine release by macrophages and suppress immune responses (Wang et al. 2014). Bacterial infections trigger intracellular signaling via MAPK cascades and NF‐κB translocation into the nucleus, leading to increased transcription of proinflammatory cytokines, including IL‐1β, IL‐6, TNFα and IFNγ. Effector proteins from bacterial pathogens can block MAPK kinase signaling and NF‐κB activation. The IpaH T3SS effector proteins of Shigella function as E3 ligases and cycle‐inhibiting factor, a T3SS effector of pathogenic E. coli, modulates or blocks degradation of IκBα to arrest host responses (Rohde et al. 2007; Ashida and Sasakawa 2015). Similarly, OspF, a T3SS effector of Shigella, or Salmonella homologs of OspF, specifically dephosphorylate MAPKs (Mattock and Blocker 2017), reducing the influx of acute inflammatory cells into infected tissues (Haneda et al. 2012; Hou et al. 2018). The adaptive immune system offers many opportunities to bacteria for subversion. Because adaptive immune responses are antigen‐specific, bacteria can evade immune effectors by changing the antigens that are expressed. Immune responses that are dependent on antigen processing can be inhibited by killing or modulating antigen‐presenting cells. Bacterial toxins can interfere with activation of lymphocytes to prevent clonal expansion. Alternatively, polyclonal stimulation of lymphocytes can inhibit pathogen‐specific responses by consuming limited resources of energy and nutrients on irrelevant activities. Modulation by bacteria of the cytokines produced by immune cells can steer immune responses away from pathways with bactericidal potential. Bacterial manipulation of host‐regulated cell‐death mechanisms, induction of epigenetic changes, and dysregulation of protein synthesis enhance pathogen survival and proliferation (Figure 5.2; Table 5.2).
5
Subversion of the Immune Response by Bacterial Pathogens
Introduction
Immune Defenses
Subversion of Innate Responses
Evasion of Antimicrobial Peptides
Impairment of Tracheal Clearance
Adhesion and Penetration of Epithelial Barriers
Evasion of Complement‐Mediated Opsonization, Chemotaxis, Killing, and Adjuvant Effects
Evasion of Complement – Physical Barriers
Subversion of the Classical Pathway
Subversion of the Alternative Pathway
Inhibition of Effects of Complement on Adaptive Immunity
Evasion of Phagocytosis
Cytotoxic Effects Against Phagocytes
Mechanism (see Figure 5.1)
Examplesa
Reduce chemotaxis of neutrophils
Bordetella (1)
Impair cell migration
Bacillus anthracis, Streptococci, Yersinia pestis (1)
Interfere with cell signaling
Helicobacter pylori, Salmonella, Y. pestis (2)
Reduce expression of proinflammatory cytokines
B. anthracis, Escherichia coli, Salmonella (1,2)
Evade uptake
Pasteurella multocida, Streptococcus suis, Y. pestis (3)
Biofilm formation
Staphylococcus epidermidis, Pseudomonas aeruginosa (3)
Interfere with receptor mediated uptake, including opsonization
Histophilus somni, Staphylococci, Streptococci (2)
Escape from phagosome into cytosol, then penetrate cell to cell
Listeria monocytogenes, Rickettsia (3)
Inhibit formation of phagolysosome
Brucella, Chlamydia, Legionella, mycobacteria (4)
Inhibit oxidative processes and microbial killing
Bordetella, H. somni, Mycoplasma bovis, mycobacteria, Staphylococcus aureus (5)
Lyse phagocytic cells
E. coli, H. somni, Mannheimia haemolytica, S. aureus (6)
Persist intracellularly
Chlamydia, mycobacteria (7)
Formation of Biofilms
Phagosomal Escape and Inhibition of Phagolysosomal Fusion
Intracellular Persistence in Activated Macrophages
Subversion of Innate Immune Receptors
Interference with Induction of Proinflammatory Responses
Subversion of Adaptive Immunity
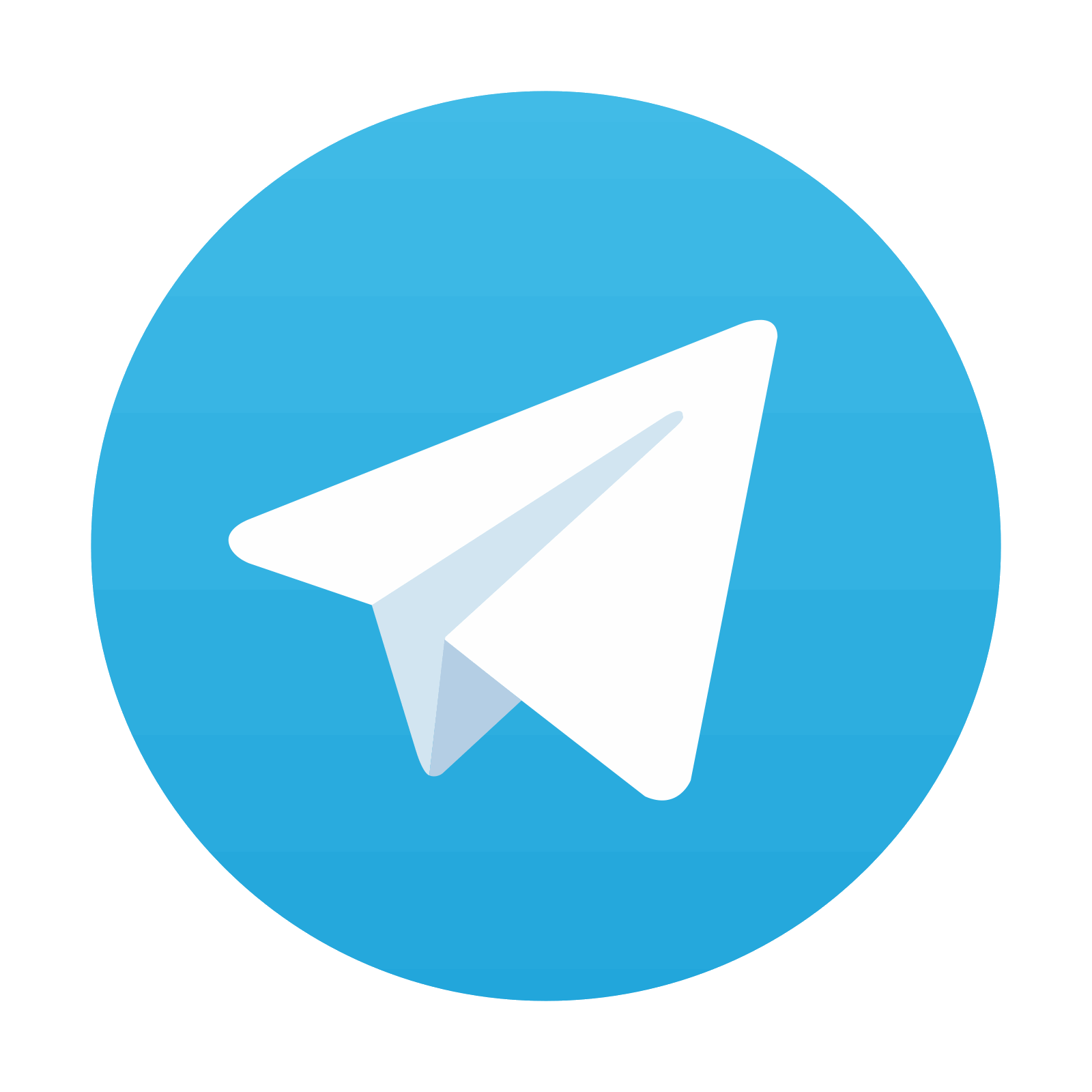
Stay updated, free articles. Join our Telegram channel
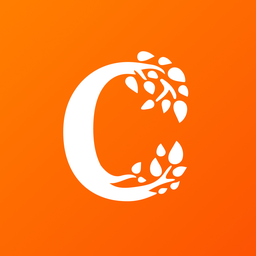
Full access? Get Clinical Tree
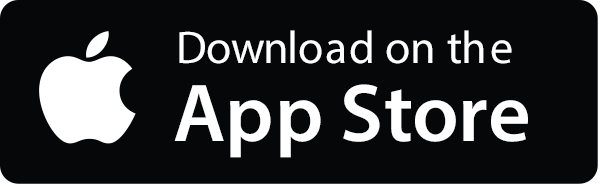
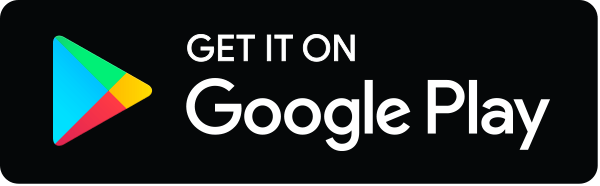