Species
Hormone assays
Reproductive stages
Samples
Citations
Giant panda
Estrogens
Estrone sulfate
Estrus
Urine
Estrone-3-glucorinade
Pregnandiol
Luteal phase
Progesterone
Luteal phase
Feces
Kersey et al. (2010)
Spectacled bear
Progesterone
Luteal phase
Urine, Feces
Dehnhard et al. (2006)
Asian black bear
Estrogens
Estrus
Feces
Chang et al. (2011)
Progesterone
Luteal phase
Sun bear
Epi-androsterone
Estrus
Feces
Schwarzenberger et al. (2004)
Pregnanediol
Luteal phase
Brown bear
Estrogens
Estrus
Feces
Progesterone
Luteal phase
Reproductive hormone metabolites have been determined to characterize reproductive cyclicity and seasonality as well as understand causes of reproductive failure in some mustelid species, including the North American river otter (Bateman et al. 2009), Asian small-clawed otter (Bateman et al. 2009), sea otter (Larson et al. 2003), black-footed ferret (Young et al. 2001) and wolverine (Dalerum et al. 2005). Specifically, analyses of gonadal hormone metabolites have revealed species differences in reproductive seasonality between the Asian small-clawed otter and North American river otter, with the latter being a seasonal breeder and females entering estrus once per year (Bateman et al. 2009). Furthermore, it has been demonstrated that the solitary North American river otter is an induced ovulator, whereas the social Asian small-clawed otter appears to be primarily a spontaneous ovulator (Bateman et al. 2009). A study in female wolverines has shown that reproductive failure may be related to low social rank and likely to be due to implantation failure independent of elevated glucocorticoid metabolites (Dalerum et al. 2005).
3.2 Semen Collection and Artificial Insemination
Semen collection in wild felids is usually performed by electroejaculation (Howard and Wildt 2009). Recently, a field-friendly technology utilizing urethral catheterization has been developed in the domestic cat (Zambelli and Cunto 2006; Zambelli et al. 2008). When compared with electroejaculation, urethral catheterization yields smaller seminal volume (67.1 ± 25.9 μl vs. 10.5 ± 5.3 μl) with high sperm cell concentration (542.9 ± 577.9 × 106/ml vs. 1,868.4 ± 999.8 × 106/ml; Zambelli et al. 2008). Furthermore, cryopreserved sperm obtained by urethral catheterization are able to fertilize conspecific oocytes at a similar percentage to electroejaculated samples (Zambelli et al. 2008). This newly established technique has been used in the African lion following medetomidine administration to yield high quality semen samples (volume [mean ± SD]: 422.9 ± 296.1 μl; motility [mean ± SD]: 88.8 ± 13.2 %; sperm concentration of 1.9 × 109/ml) (Lueders et al. 2012).
Recovery of sperm cells from the epididymis post castrationem or post mortem has also been reported in wild carnivores (Anel et al. 2011; Jewgenow et al. 1997). This technology allows the rescue of gametes from genetically under-represented animals that undergo castration for medical reasons or die unexpectedly (Jewgenow et al. 1997; Johnston et al. 1991). In the domestic cat, seminal characteristics and fertilizing capacity of epididymal samples are comparable to those of ejaculated samples (Filliers et al. 2010). Due to the rarity of conspecific gametes, fertility of epididymal sperm obtained from wild felids has only been examined in vitro using heterologous fertilization with domestic cat oocytes (Ganan et al. 2009; Jewgenow et al. 2011). It is worth mentioned that heterologous in vitro fertilization using domestic cat oocytes has also been used to assess the fertility of sperm obtained from other carnivore species including the giant panda (Spindler et al. 2006) and to differentiate binding/fertilizing capacity between individual males and species (Baudi et al. 2008; Niu et al. 2006). To-date, there are no reports on collection of epididymal sperm from wild canids (Silva et al. 2004; Thomassen and Farstad 2009). However, a study in the domestic dog has reported birth of puppies born from a female inseminated with fresh epididymal sperm from a benign prostatic hyperplasia dog (Klinc et al. 2005). Furthermore, it has been shown that in vivo fertility of cryopreserved dog epididymal spermatozoa is lower than that of ejaculated samples, although no differences in sample quality post-thaw are observed (Thomassen and Farstad 2009). Recently, it has been reported that epididymal sperm from a Cantabrian brown bear (Ursus arctos) cryopreserved in a 430 mOsm Test-Tris Fructose + 4 % glycerol + 15 % egg yolk exhibited close to 70 % motility after freezing and thawing (Anel et al. 2011).
Artificial insemination, especially in combination with sperm cryopreservation, is a valuable tool for managing threatened wildlife populations because this strategy eliminates transportation of animals from different locations for breeding, and allows re-infusion and dissemination of valuable genes even after death of the sperm donor. Despite these great potentials, the practical application of AI to the conservation of carnivores is still quite limited (Swanson 2006). Successful AI has been reported in only a few wild carnivore species to-date using both surgical and non-surgical techniques, with some progress during the past 10 years. Summary of felid species in which live offspring have been produced by AI (fresh or frozen semen) has recently been reported elsewhere (Howard and Wildt 2009). Table 10.2 summarizes successful AI in canids, ursids and mustelids.
Table 10.2
List of canids, ursids and mustelids that offspring have been produced by artificial insemination
Species | Gonadotropin | Sperm deposition | Sperm type | No pregnancies | References |
---|---|---|---|---|---|
Canids | |||||
Gray wolf | GNRH agonist | TVI/TCI | Fresh | 1/3 (33.3 %) | Asa et al. (2006) |
Gray wolf | Frozen | 1 | Seager et al. (1975) | ||
Mexican gray wolf | N/A | IUI | Fresh | 3/3 | Thomassen and Farstad (2009) |
Red wolf | natural | LUI | Fresh | 1 L produced | Goodrowe et al. (1998) |
Red fox | N/A | N/A | Fresh/Frozen | 80 % (fresh sperm) | Farstad (1998) |
Blue fox | N/A | N/A | Fresh/Frozen | 80 % | |
Ursids | |||||
Giant panda | Natural | TVI | Frozen | 5/14 | Huang et al. (2012) |
Giant panda | Natural | Fresh | Masui et al. (1989) | ||
Mustelids | |||||
European ferret | Natural estrus/hCG | LUI | Fresh | 17/24 (70.8 %) | Wildt et al. (1989) |
European ferret | Natural estrus/hCG | LUI | Frozen | 7/10 (70.0 %) | Howard et al. (1991) |
Black-footed ferret | Natural | LUI | Fresh/Frozen | Howard and Wildt (2009) |
Most recently, laparoscopic oviductal AI has been developed in felids (Swanson 2012). The utilization of this technique overcomes challenges associated with the complexity of the female reproductive tract and poor seminal quality observed in many felid species. To-date, offspring have been produced after laparoscopic oviductal AI in the ocelot, Pallas’ cat and domestic cat (Swanson 2012). Furthermore, an ultrasound guided trans-cervical insemination in which sperm are non-surgically deposited deep into the cervix has also been developed and successfully applied in the lion, cheetah and Amur leopard (Goeritz et al. unpublished data; Goeritz et al. 2012).
For canids, with the exception of the domestic dog and the farmed fox species, semen collection is normally performed by electroejaculation (Asa et al. 2007a, b; Goodrowe et al. 1998; Johnston et al. 2007; Minter and DeLiberto 2008; Songsasen et al. 2013). A major challenge in semen collection in canids is contamination of urine in semen samples obtained from electroejaculation (Platz et al. 2001). The presence of urine alters osmolarity and pH of the seminal sample and increases the proportions of sperm with bent and coiled tail as well as decreasing motility (Platz et al. 2001) and may affect the susceptibility of sperm to osmotic stress during the cryopreservation process (Johnson 2012). A recent study has demonstrated that maned wolf ejaculates can be obtained using digital stimulation (Teodoro et al. 2012) with seminal characteristics similar to those obtained by electroejaculation (Johnson 2012; Songsasen et al. 2013).
Seminal characteristics vary among wild canid species (Table 10.3), with the maned wolf having the lowest seminal quality (low sperm output and high percentage of structurally abnormal cells (Comizzoli et al. 2009; Johnson 2012; Teodoro et al. et al. 2012). Ejaculate traits of free ranging maned wolves have also been investigated, showing similar comparable seminal traits to those of captive individuals with a high proportion of structurally abnormal spermatozoa (Songsasen et al. 2013). The high proportions of abnormal spermatozoa observed in both captive and wild wolves may be linked to low genetic diversity (nucleotide diversity [π] = 0.0013) as the result of genetic bottle neck during or at the end of the last glacial maximum (Prates Junior 2008). Low gene diversity has been shown to be associated with poor seminal quality in other carnivore species, including the Florida panther (Felis concolor coryi; Facemire et al. 1995), cheetah (Fitzpatrick and Evans 2009), black-footed ferret (Fitzpatrick and Evans 2009) and gray wolf (Asa et al. 2007a). Unlike wild felids, artificial insemination has not been widely used in wild canids, due to the lack of knowledge on species’ reproductive biology and the challenges in predicting ovulation onset and effectively manipulating the female reproductive cycle (Thomassen and Farstad 2009).
Table 10.3
Seminal traits of the wild canids
Species | Volume (ml) | Concentration (×106 sperm/ml) | Motility (%) | Morphologically normal sperm (%) | Citations |
---|---|---|---|---|---|
Gray wolfa | 1.7 ± 0.2 | 290.8 ± 53.5 | 91.7 ± 1.5 | N/A | Mitsuzuka (1987) |
Coyote | 1.7 ± 0.4 | 549.2 ± 297.7 | 90.4 ± 4.5 | 78.0 ± 13.5 | Minter and DeLiberto (2008) |
Red wolf | 4.7 ± 0.7 | 146.5 ± 25.7 | 71.2 | 80 % | Goodrowe et al. (1998) |
African wild dog | 0.6 ± 0.1 | 212.3 ± 87.3 | 69.5 ± 3.3 | N/A | Johnston et al. (2007) |
Maned wolf | 2.0 ± 0.6 | 29.5 ± 9.3 | 65.0 ± 6.1 | 50.1 ± 8.1 | |
Maned wolfa | 1.3 ± 0.14 | 56.8 ± 7.8 | 76.1 ± 2.8 | 36.5 ± 3.4 | Teodoro et al. (2012) |
Blue fox | 0.4 ± 0.3 | 491.8 ± 372.1 | N/A | 89.9 ± 4.4 | Stasiak et al. (2008) |
With the exception of the giant panda, little progress has been made in establishing AI in ursids, although this technology will greatly benefit captive management of Malaysian sun bear and spectacled bear populations. Successful pregnancies of the giant panda following AI was achieved in the 1980s (Masui et al. 1989; Moore et al. 1984). Because the conception rate after AI with fresh semen is similar to that after natural mating, this technology is now routinely used in the captive management of this species (Hori et al. 2006; Huang et al. 2012). Furthermore, it has been shown that there are no differences in the ability of fresh and cryopreserved giant panda sperm to undergo decondensation after exposure to cat oocyte ooplasm (Spindler et al. 2006). To-date, live offspring have been produced after AI with cooled and frozen-thawed sperm (Pukazhenthi and Wildt 2004). Due to similarities in morphology of the reproductive organs, it may be possible that instruments used for giant panda AI can be adapted to other bear species (Knauf 2006). Semen collection, seminal analysis and sperm cryopreservation procedures have been reported in other bear species (Chen et al. 2007; de Paz et al. 2012; Ishikawa et al. 2002).
Semen samples have been collected by electroejaculation in some mustelids, including North American river otter (Bateman et al. 2009), black-footed ferret, Siberian polecat and domestic ferret (Howard et al. 1991; van der Horst et al. 2009). The timing of the breeding season has been shown to influence seminal quality in ferrets (van der Horst et al. 2009). Specifically, there is a higher percentage of structurally abnormal sperm in samples collected at the beginning of a breeding season than those collected during the peak of the reproductive season. Although low seminal output is observed in the black-footed ferret compared with the domestic ferret and Siberian polecat, fresh sperm from the three species exhibit similar percentages of normal morphology, sperm motion characteristics and responses to cryopreservation (van der Horst et al. 2009), indicating that the two common ferret species are suitable models for developing reproductive technologies for their endangered counterpart. To-date, offspring have been produced from artificial inseminations with fresh and frozen semen in two mustelid species, including the black-footed ferret (Table 10.2; Howard and Wildt 2009; Howard et al. 1991).
3.3 In Vitro Oocyte Maturation/Fertilization and Embryo Transfer
The abundant supply of domestic cat ovaries from veterinary and spay clinics have made this species a practical and valuable model for reproductive studies in wild counterparts (Pope et al. 2006; Comizzoli et al. 2010). Extrapolation of reproductive technologies developed in the domestic cat has yielded encouraging outcomes in several wild felids. Currently, in vitro fertilization of ovarian oocytes (in vivo or in vitro matured) obtained from live animals (ovum pick-up or ovariohysterectomy) or after death of a female has been reported in several wild felid species. These include the leopard cat (Felis bengalensis; Goodrowe et al. 1989), tiger (Donoghue et al. 1990), Siberian tigers (P. tigris altaica; Crichton et al. 2003), cheetah (Crosier et al. 2011; Donoghue et al. 1992), Indian desert cat (Felis silvestris ornate; Pope et al. 1993), jungle cat (Felis chaus; Pope et al. 1993), black-footed cat (Felis nigripes; Pope et al. 1993), fishing cat (Felis viverrinus; Pope et al. 1993), lion (Armstrong et al. 2004), African wild cat (Pope et al. 2006), ocelot (Swanson 2006), sand cat (Swanson 2012) and caracal (Pope et al. 2006). Among these, live offspring have been produced after transferring fresh or frozen embryos in the Siberian tiger (Donoghue et al. 1993), Indian desert cat (Pope et al. 1993), African wildcat, Caracal (Pope et al. 2006), ocelot (Swanson 2006), sand cat (Swanson 2006, 2012) and black-footed cat (Pope et al. 2012a). Intracytoplasmic sperm injection (ICSI) has also been attempted in wild felids, and high cleavage rates were reported (lion: 60 %, fishing cat: 70 %), but transfer of pre-implantation stage embryos did not result in a pregnancy (Pope et al. 2006). Although live cubs have been produced in numbers of wild felids, in vitro embryo production has not been widely used into captive breeding program mainly due to several reasons. First, there is limited information on species-specific reproductive endocrinology, gamete biology and embryogenesis. The second is the complexity of the procedure and the need for specialized equipment and facilities to recover oocyte, perform in vitro fertilization and culture embryos. The third reason is limited availability of developmentally competent oocytes, especially of poor health/aging females. Because ovaries are required to be transported to a laboratory, it is essential to optimize storage condition that allows the maintenance of gamete quality for at least 24 h. Unlike the male, where ICSI can be applied to overcome poor quality samples (low motility; Ringleb et al. 2010), there is no alternative method to circumvent this challenge for the female gamete.
In the domestic dog, in vitro maturation and fertilization has been far from being successful, due to the unique reproductive (prolonged anestrus) and oocyte (protracted oocyte maturation within the oviduct) biology (Songsasen and Wildt 2007). Therefore, no or little progress has been made regarding in vitro production of embryos in wild canids. The first report on in vitro fertilization of in vitro matured oocytes was in the domestic dog by Mahi and Yanagimachi (1976), although embryonic development was not reported. Since then, several studies have been conducted to examine the variety of micro-environmental factors (e.g., hormones, energy substrates and culture media) influencing developmental competence of dog oocytes (Songsasen and Wildt 2007), but still with limited success in oocyte and embryo development outcomes (Luvoni et al. 2005; Songsasen and Wildt 2007). To date, there is only one report demonstrating the production of a single blastocyst from in vitro fertilization (Otoi et al. 2000), and one non-full term pregnancy after transferring close to 100 in vitro derived presumptive zygotes in the domestic dog (England et al. 2001). In vitro maturation and fertilization have also been conducted in silver (red) fox with minimal success (Feng et al. 1994). In that study, only 19 % of oocytes reached the metaphase I/metaphase II after 48 h in vitro culture, and sperm penetration was observed in 18 % of cultured oocytes (Feng et al. 1994); no embryonic development was recorded in this study. In vitro fertilization of in vivo matured oocytes has been reported in the blue fox (Farstad et al. 1993). In that study, 5 of 13 oocytes (38.4 %) obtained 6 days post LH developed into 2-cell stage post-insemination, and one embryo reached the morula stage.
In bears, very little efforts have been made to establish protocols for in vitro production of embryos, although some sporadic attempts have been performed. Ovarian oocytes recovered from American black bear, sun bear, sloth bear (Johnston et al. 1994) and giant pandas (Zhang et al. 1998) are able to complete nuclear maturation in vitro. More recently, it has been shown that 50 % of brown bear oocytes complete nuclear maturation after 48 h in vitro culture (Yin et al. 2007). Electrical activation of the in vitro cultured oocytes has resulted 31 % of activated gametes developing into 2–4 cell stage (Yin et al. 2007). Recovery of in vivo produced embryos during diapause has been reported in Brown bear (Tsubota et al. 1991). Furthermore, Boone et al. (1999) reported the live birth of an American black bear cub after non-surgical embryo collection following by laparoscopic embryo transfer. In that study, the delayed implantation allowed successful development of the embryos despite a substantial asynchrony between the donor and the recipient (Boone et al. 1999).
In vitro maturation of mustelid oocytes has been conducted only in the domestic ferret. Supplementing culture media with gonadotropins has been shown to enhance nuclear maturation capacity (Li et al. 2002; 70 % after 24 hours IVM compared with 30 % of no hormone treatment). Oocytes (43 %) matured under this condition were able to develop to the blastocyst stage after in vitro activation using cycloheximide and 6-dimethylaminopurine followed by electrical stimulation (Li et al. 2002).
To-date, in vitro fertilization has not been reported in mustelids. However, there have been a few reports on in vitro culture of in vivo produced embryos in the domestic ferret (Li et al. 2001), European polecat (Lindeberg and Jarvinen 2003), stoat (Amstislavsky et al. 2012) and American mink (Moreau et al. 1995). For the ferret and European polecat that do not exhibit delayed implantation, blastocyst development after in vitro culture has been observed (Li et al. 2001; Lindeberg and Jarvinen 2003), and the production of live offspring after embryo transfer has been reported in the former (Li et al. 2001). For stoat, a species with obligate delayed implantation, 1 to 4 cells stage embryos are able to develop to the blastocyst stage; however, diapausing embryos fail to develop in culture (Amstislavsky et al. 2012). Co-culture with Buffalo rat liver cell has been shown to enable American mink diapausing embryos to develop into hatch blastocysts (Moreau et al. 1995).
3.4 Preservation of Female Germplasm
3.4.1 Oocyte and Embryo Cryopreservation
Genetic resource banking is defined as the storage of gametes (sperm and oocytes) and embryos with the deliberate intention to use these valuable biomaterials to maintain gene diversity of threatened populations (Holt and Pickard 1999). In combination with reproductive technologies, it allows re-infusion and dissemination of valuable genes independently of time and geographical locations. In addition to gametes and embryos, somatic cells and tissues have been considered as valuable biological resource because of their broad applications in genetics, toxicology and epidemiology (Leon-Quinto et al. 2008). Furthermore, with the rapid progress in somatic cell nuclear transfer technologies (Gomez et al. 2009; Kim et al. 2007), preservation of somatic cell lines may offer future reproductive opportunities for animals whose viable gametes cannot be obtained (Leon-Quinto et al. 2008; Lermen et al. 2009).
Cryopreservation of feline oocytes is still considered to be an experimental technique (Luvoni 2006), although births of live kittens produced from vitrified domestic cat oocytes have been reported (Pope et al. 2012b; Tharasanit et al. 2011). Despite this success, cryopreservation of immature and mature cat oocytes is not proven to be a reliable tool; the cleavage rate of frozen-thawed domestic cat oocytes is less than 25 % (Cocchia et al. 2010; Comizzoli et al. 2006; Merlo et al. 2008; Tharasanit et al. 2011). Yet, a recent study has offered a novel alternative through germinal vesicle preservation (Comizzoli et al. 2011). In that study, germinal vesicles recovered from fresh or vitrified oocytes were transferred into the cytoplasm of fresh Grade I gametes; the reconstructed oocytes from both groups were able to complete nuclear maturation (80 %) and developed to blastocyst after IVF at a similar capacity (15 %). To date, there are no reports on oocyte cryopreservation in wild felids.
For canids, advances in oocyte cryopreservation have been reported during the past 5 years (Abe et al. 2008; Boutelle et al. 2011; Turatham et al. 2010; Zhou et al. 2009). By using the open-pulled straw technique, the percentage of vitrified-warmed dog oocytes completing nuclear maturation was similar to that of fresh controls, although more cryopreserved gametes were arrested at the GV stage than those of the fresh counterparts (Turatham et al. 2010). Blue fox oocytes vitrified using the two-step open-pulled straw method also developed to the MII at the similar percentage to those of the fresh control (Zhou et al. 2009). Recently, it has been shown that dog and Mexican gray wolf oocytes maintained viability (based on propidium iodide staining) after vitrification using the cryotop technique (Abe et al. 2008; Boutelle et al. 2011).
Successful embryo cryopreservation in canids either by vitrification (Abe et al. 2011) or slow freezing method (Guaitolini et al. 2012) has recently been reported in the domestic dog. It has been shown that there appears to be a stage-dependency in the susceptibility to vitrification (Abe et al. 2011). Specifically, blastocysts are more sensitive to vitrification than those at the earlier stages of development (one-cell to morula stages) (Abe et al. 2011). Transferring of vitrified-warmed dog embryos has resulted in the birth of live offspring (7 pups/77 transferred embryos; 9.1 %; Abe et al. 2011). Dog blastocysts also have been cryopreserved using a slow freezing method (Guaitolini et al. 2012; Kim et al. 2002). Although cryopreserved blastocysts maintain viability in vitro for 6 days, transfer of frozen-thawed embryos results in no offspring (Guaitolini et al. 2012; Kim et al. 2002).
3.4.2 Ovarian Tissue Cryopreservation
The ability to successfully cryopreserve ovarian tissues has potential importance for the genetic management of rare species, especially carnivores, as the majority of these animals are strictly seasonal breeders (i.e., developmentally competent gametes can be recovered only during reproductively active period; Comizzoli et al. 2012). Viable banks of tissues can be used in combination with in vitro culture, allo- or xenografting followed by IVM/IVF to produce developmentally competent embryos (Agca et al. 2009; Cleary et al. 2003; Comizzoli et al. 2012; Fassbender et al. 2007; Jewgenow and Paris 2006; Kim et al. 2009; Newton et al. 1996; Paris et al. 2004). This technology is also useful in cases of unexpected death of pre-pubertal individuals. The challenges in establishing cryopreservation procedures for ovarian tissue are the complexity of gonadal tissue structure, cell heterogeneity and the lack of basic cryobiological and developmental knowledge. Within the ovarian cortex, there are thousands of primordial follicles containing oocytes at their least differentiated stage, which appear to be relatively resistant to vitrification procedure (Comizzoli et al. 2012). Ovarian tissue cryopreservation has been conducted in the domestic cat (Bosch et al. 2004; Comizzoli et al. 2012). It has been demonstrated that more cat follicles within ovarian cortices maintain structural integrity after vitrification in a solution containing 15 % ethylene glycol + 15 % DMSO + 0.5 M sucrose than after being cryopreserved using a standard slow-cooling method (Comizzoli et al. 2012). To-date, ovarian cortices of the black-footed ferret, cheetah and clouded leopard have been vitrified using the method developed in the domestic cat; the vitrified tissues maintained structural integrity, although the developmental potential of cryopreserved follicles has not been examined (Comizzoli et al. 2012). Recently, Wiedemann et al. (2012) have demonstrated follicular development in lions after ovarian tissue cryopreservation using a slow cryopreservation protocol following xenografting into immunodeficient mice. Despite some progress in the cryopreservation of ovarian tissue, it has been demonstrated that isolated small preantral cat follicles are rather susceptible to cryopreservation with only 10 % remaining structurally intact and physiologically active after thawing (Jewgenow et al. 1998).
3.5 Somatic Cell Nuclear Transfer
Since the birth of the sheep Dolly in the late 1990s (Campbell et al. 1996), much progress has been made in establishing somatic cell nuclear transfer (SCNT) technology in a wide-variety of species, including carnivores. This reproductive technology is often suggested to have potential for conservation of endangered wildlife, especially when it aims at recovering genes from under-represented individuals (Holt et al. 2004). To-date, SCNT studies focusing on establishing cell line and cell cycle synchronization have been conducted in both domestic and wild carnivores (de Barros et al. 2010; Koo et al. 2009; Tao et al. 2009; Verma et al. 2012; Wittayarat et al. 2012). Live births also have been reported in the domestic cat (Yin et al. 2005; Yin et al. 2008), dog (Hossein et al. 2009a, b; Jang et al. 2010; Jang et al. 2008) and gray wolf (Kim et al. 2007; Oh et al. 2008). Cats and dogs produced by SCNT have been shown to exhibit normal health and reproductive potential (Choi et al. 2010; Park et al. 2010). However, the practical application of SCNT to wildlife conservation is still limited because of its low success rate of <0.1–6 % of reconstructed embryos developing to live offspring, due to abnormal nuclear reprogramming of the transplanted somatic cells (Holt et al. 2004; Loi et al. 2011).
An additional limiting factor in the implementation of SCNT technology for endangered species is the scarcity of conspecific oocytes and embryo transfer recipients. It has been suggested that interspecific (or intergeneric) SCNT which involves transferring cell of one species into enucleated oocytes of another species, and then establishing pregnancy by interspecific embryo transfer, can circumvent this challenge (Loi et al. 2011). While transferring wild felid embryos into domestic cat recipients has resulted in births of live offspring (Pope et al. 1993, 2012a, b), embryos produced by interspecific SCNT have limited developmental capacity, due to abnormal reprogramming of donor cells (Gomez et al. 2012; Imsoonthornruksa et al. 2012; Lee et al. 2010; Thongphakdee et al. 2010). Epigenetic modifications of donor somatic cells have been shown to enhance in vitro development of reconstructed embryos, and regulated the expression of pluripotent genes, but this strategy does not result in full-term pregnancy in the leopard cat (Lee et al. 2010) and black-footed cat (Gomez et al. 2011, 2012). To-date, births of live offspring produced by interspecific SCNT have been reported in the African wild cat (1 % success rate; Gomez et al. 2004) and sand cat (0.9 %; Gomez et al. 2008). Intergeneric SCNT also has been attempted in the giant panda (Chen et al. 2002). Giant panda cell nuclei were transplanted into rabbit oocytes and development of reconstructed embryos up to blastocyst stages was demonstrated (Chen et al. 2002). Furthermore, co-transferring of giant panda-rabbit reconstructed embryos with cat-rabbit cloned counterparts resulted in implantation of the former in the cat uterus (Chen et al. 2002).
Recently, alternative approaches have been explored to overcome the challenge associated with the scarcity of conspecific oocytes for SCNT (or IVM/IVF/ICSI). These include recovery of gametes from ovarian tissues transplanted to immunodeficient hosts (Bosch et al. 2004; Fassbender et al. 2007; Wiedemann et al. 2012) and generating in vitro-derived gametes from gonadal stem cells (Gomez et al. 2010; Kashir et al. 2012; Ko et al. 2012). Although both technologies are in a very early stage in carnivores (Gomez et al. 2010), the feasibility of these approaches has already been demonstrated in other mammalian species, including the mouse and human (Hayashi, et al. 2012; Li et al. 2010). Similar approaches have also been investigated for in vitro production of male gametes in many mammalian species, including the domestic cat and dog (Kim et al. 2006; Kim et al. 2008; Powell et al. 2012; Travis et al. 2009).
4 Captive Breeding Programs for Conservation, Linking Ex Situ: In Situ Conservation in Critically Endangered Carnivores
For many critically endangered carnivore species (or subspecies), e.g. the Iberian lynx, Amur leopard, black-footed ferret, the giant panda, or red wolf, captive breeding programs have been considered as an integral part of the conservation action plans. As mentioned in the Introduction, ex situ populations mainly serve as (1) a genetic repository stock for reintroduction to bolster the numbers of the wild counterpart projects (Calzada et al. 2009; Christie 2009; Vargas et al. 2008) and (2) research resources to better understand species’ biology. Although reintroduction programs have been launched in many carnivores, including the black-footed ferret, gray wolf, African lion, African wild dog, tiger, snow leopard, leopards, jaguars and cheetah (for review; Hayward and Somers 2009), captive breeding programs that take advantage of advanced reproductive sciences and technologies to genetically manage the population are rarely found.
The Conservation programs for the black-footed ferret (Comizzoli et al. 2009), Iberian lynxes (Vargas et al. 2008, 2009) and giant panda may serve as examples of how reproductive science contributes to the conservation of a species. Because there is a chapter in this book dedicated specifically to the Black-footed ferret, here, we will only focus on how reproductive sciences assist in the conservation program of the Iberian lynx and the giant panda.
Since the inauguration of the Iberian lynx captive breeding program in Spain (ILCBP) in 2002, research has been conducted to advance the understanding of reproductive biology of this species. So far, the reproductive biology of this critically endangered species has been fully characterized (Goeritz, et al. 2006; Goeritz et al. 2009; Jewgenow et al. 2006; Pelican et al. 2006), semen and somatic cells regularly obtained for genome banking (Ganan et al. 2009; Leon-Quinto, et al. 2008), and some important breeding management tools, including pregnancy diagnosis have been developed and implemented (Braun et al. 2009; Finkenwirth et al. 2010). Because of this monumental effort, ILCBP has successfully propagated lynxes ex-situ and reintroduced the first captive born individuals to the wild in 2010, only 8 years after the inauguration of the program. Currently, ILCBP is continuing captive breeding efforts to produce healthy individuals for reintroduction programs (Vargas et al. 2008).
The giant panda, a bear that rely mainly on bamboos as a diet is listed as endangered by the IUCN and <2,000 individuals are estimated to remain in the wild (IUCN 2013). Because of the unstable status of the wild giant panda, tremendous efforts have been placed on establishing a self-sustaining captive population this species. Since the beginning of this century, reproductive and health studies have been conducted with the goal of overcoming poor reproduction and health of ex situ individuals to eliminate the need for removing wild counterparts (Wildt et al. 2003). Reproductive endocrinology of male and female giant pandas has been extensively characterized (Aitken-Palmer et al. 2012; Kersey et al. 2010). Furthermore, reproductive technologies such as artificial insemination and semen cryopreservation have been successfully established, and are often incorporated into breeding programs (Huang et al. 2012; Wildt et al. 2003). This multidisciplinary effort has resulted in the tremendous success in breeding and maintaining giant panda ex situ; the ex situ population has now increased from 104 in 1996 to >300 adults during the past 15 years (Wildt et al. 2007). During the same period, efforts at protecting species’ habitat have also been emphasized, and the number of giant panda reserves have increased from 13 in 1989 to >40 today (Wildt et al. 2007). Due to these successes, there is a plan to re-introduce captive born individuals to increase the numbers of wild population in the near future (Gong et al. 2012).
5 Summary
Advances in reproductive studies have improved our understanding about reproductive mechanisms that in turn allows us to establish tools to genetically manage carnivore populations ex situ (Comizzoli et al. 2009; Howard and Wildt 2009; Pukazhenthi and Wildt 2004; Vargas et al. 2009). While reproductive science has greatly contributed to the management of captive breeding programs of some carnivore species, including the Iberian lynx, black-footed ferret and giant panda, several reproductive technologies (especially for preserving female genetics) are still considered experimental and far from being included to the routine practices (Wildt et al. 2010), mainly due to the lack of basic knowledge on species’ reproduction. As of the beginning of the twenty-first century, only 2 % of mammals in the planet are considered ‘well studied’ (Wildt et al. 2010). Obviously, domestic species (i.e., cat, dog and ferret) have served as valuable models for establishing reproductive technologies in wild carnivores. Yet, there are still needs for species-specific research due to enormous diversity in reproductive biology among species, even within the same phylogenetic clade (Wildt et al. 2010). Specifically, high priority research should include characterizing reproductive traits (cyclicity and seasonality) especially in under-studied species. Furthermore, it is crucial for us to enhance the ability to manipulate female reproductive cycles, especially of embryo recipients. Finally, fundamental research should be conducted in parallel to advance our understanding of molecular and cellular mechanisms regulating gamete and embryo development, information that is crucial for successful implementation of ‘high-tech’ reproductive technologies to wild carnivores.
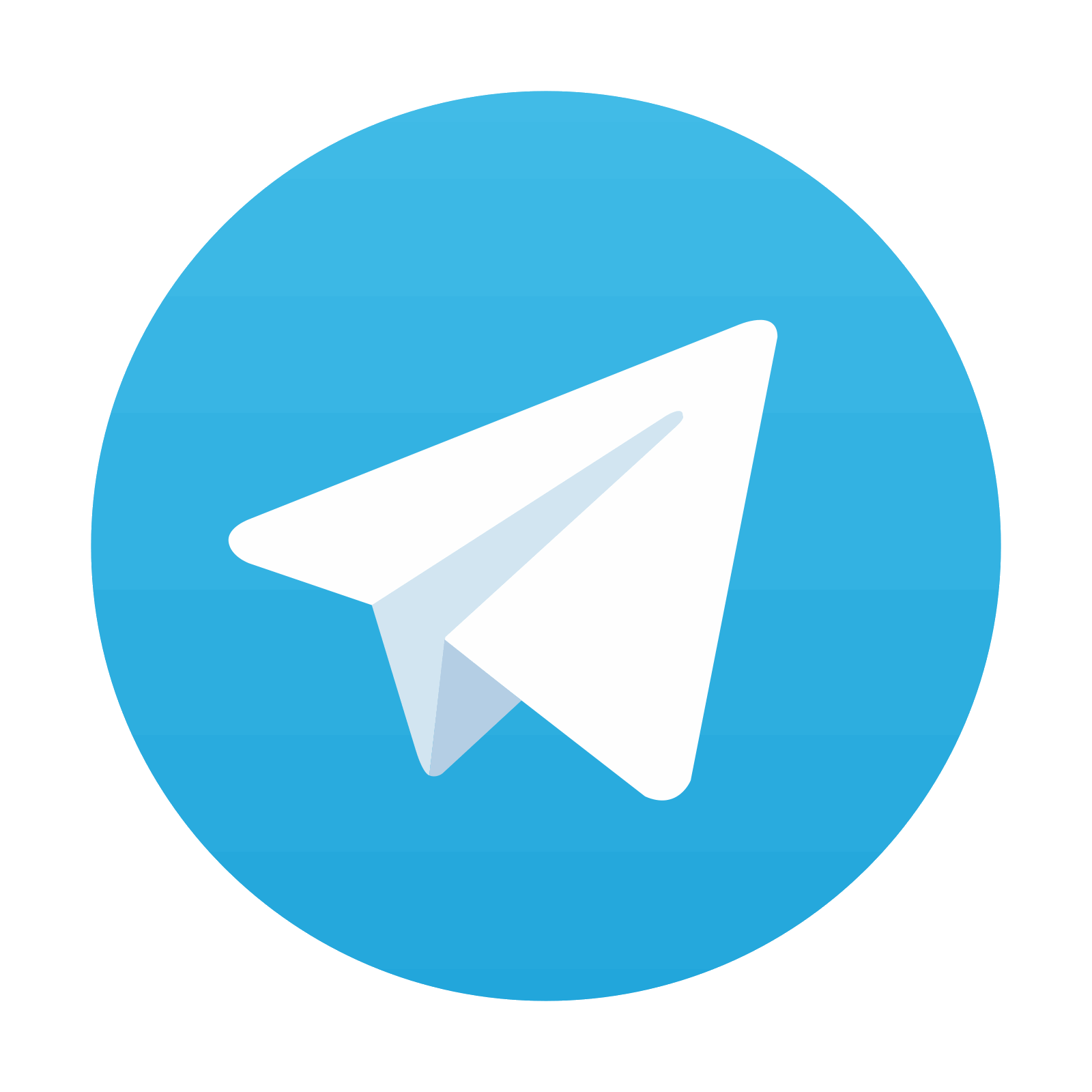
Stay updated, free articles. Join our Telegram channel
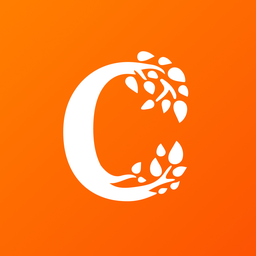
Full access? Get Clinical Tree
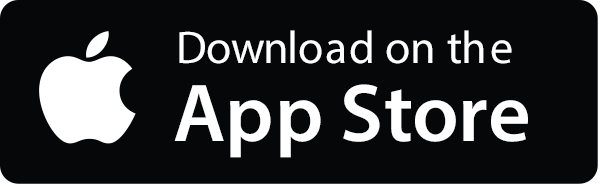
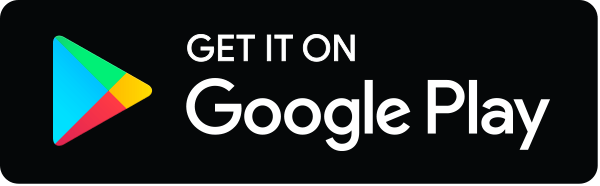