CHAPTER 6 Magnetic resonance imaging (MRI) is the imaging modality of choice for the diagnosis of most neurologic diseases in human and veterinary patients. While an in-depth review of MR physics is beyond the scope of this chapter, an overview of basic principles and sequences will be provided to facilitate understanding of clinical applications and interpretation. In general, any element with either an odd number of protons or an odd number of neutrons has a nuclear magnetic dipole moment and may therefore be suitable for MR imaging or spectroscopy. Hydrogen is the optimal element for MR imaging as (1) it is the most common element in the body, (2) its nucleus consists of a single proton and has the strongest magnetic dipole moment of any element suitable for MR imaging, and (3) most pathologic processes affecting the central nervous system (CNS) result in alteration of content, distribution, and ambient environment of hydrogen protons facilitating differentiation of diseased from normal tissue. This overview will focus on MR imaging of hydrogen protons. Hydrogen protons in tissues are not static but spin around their axes, generating their own micromagnetic environments. In the absence of an external magnetic field the magnetic moments of the spinning protons are randomly oriented. However, when brought into a strong external magnetic field (i.e. an MR scanner), they rearrange under its influence. The magnetic field strength is denoted by the unit “tesla” (T). The strength of clinically used MR scanners ranges from approximately 0.2T (low-field) to 3T (high-field); higher-strength scanners (7–21T) are at this point limited to research institutions. The alignment of individual protons may be parallel or antiparallel with the external magnetic field. A slight majority of protons will align with the magnetic field, generating a magnetic vector (“net magnetization vector”) which is utilized during MR imaging. The main magnetic field is denoted by B0, the tissue magnetization vector by M0. As long as M0 is parallel with the much stronger B0 it cannot be easily separated out and cannot be used for imaging. The goal of MR imaging is to manipulate tissue magnetization in a way that it can be distinguished from the external magnetic environment. In addition to a spinning motion around their individual axes, hydrogen protons wobble (or precess) under the influence of B0, similar to a spinning top wobbling under the influence of gravity. The precession frequency of the spinning protons is dependent on the strength of the external magnetic field and is described by the Larmor equation: where: ω0 = Larmor or resonant frequency γ = gyromagnetic ratio specific for each MR active nucleus (42.56 MHz/T for hydrogen) B0 = external magnetic field strength. In order to manipulate tissue magnetization so it can be separated from the main magnetic field, radiofrequency (RF) pulses are applied. Once the nuclei are exposed to an RF pulse exactly matching their precessional frequency they gain energy and start resonating. As a result of the energy gain some protons change their alignment with the magnetic field, causing the magnetic net vector to move away from B0, or “flip.” The most common flip angle of the tissue magnetization vector is 90° used in spin echo sequences (see below). Hydrogen protons flipped into this “transverse plane”—which is in perpendicular orientation to the main magnetic field (the “longitudinal plane”)—continue to precess. According to Faraday’s law, any change in the magnetic environment of a coil of wire will cause an electric signal. Strategic placement of receiver coils in the MR unit allows detection and measurement of magnetization in the transverse plane, which is the basis of image formation in MRI (Fig. 6.1). Figure 6.1 Schematic illustration of the alignment of the net vector (tissue magnetization) before (A) and after (B) application of a 90° RF pulse. (A) The net vector is aligned parallel to the main magnetic field (B0) and cannot be measured. (B) After application of the 90° pulse the net vector is oriented perpendicular to the main magnetic field, and a current (signal) is induced in the receiver coil. (The Ohio State University. Reproduced with permission.) After the RF pulse is switched off, the signal induced in the receiver drops off rapidly due to two concurrent processes: Figure 6.2 Schematic illustration of T1 relaxation. (A) Situation before the 90° pulse. The magnetic vector is aligned with the main magnetic field. (B) After the 90° pulse the vector is in the transverse plane. (C, D) The protons realign with the magnetic field. (The Ohio State University. Reproduced with permission.) Figure 6.3 Schematic illustration of T2/T2* relaxation. (A) Situation before the 90° pulse. The magnetic vector is aligned with the main magnetic field. (B) After the 90° pulse the vector is in the transverse plane, the protons form a unified front, and the MR signal is strongest. (C, D) The precessing protons lose coherence, and the strength of the MR signal decreases. This occurs due to interference of individual protons with each other (T2 relaxation) as well as influence of external field inhomogeneities (T2* relaxation). (The Ohio State University. Reproduced with permission.) Three important tissue parameters for MR imaging include T1- and T2-relaxation times and proton density (PD), i.e. the actual amount of hydrogen protons in a certain tissue volume. The goal of MR imaging is to translate these inherent tissue differences into image contrast, which is accomplished by using different MR sequences. These include T1-W, T2-W and proton density (PD) weighting, which are the most basic but also the most commonly used MRI sequences. Each SE sequence starts with a 90° RF pulse followed by a 180° pulse applied exactly halfway between the initial 90° pulse and the generation of the signal (echo) (Fig. 6.4). The 180° pulse is applied to cancel out external magnetic field inhomogeneities. It essentially reverses any effects an external disturbance (e.g. a nearby flowing vessel or paramagnetic methemoglobin in a hematoma) will have on proton alignment and resultant tissue signal. The small interactions occurring between individual protons and contributing to signal loss in the transverse plane cannot be reversed, resulting in true T2 relaxation contributing to image contrast. As the MR signal generated during a single episode of proton excitation is too small to create an image, the process is repeated many times until enough data have been collected. The time between the 90° pulse and the echo is called “time of echo” (TE) and the time between successive 90° pulses is called “time of repetition” (TR). The length of TR and TE determines weighting of an SE sequence (Table 6.1). Table 6.1 Influence of acquisition parameters on image contrast in a spin echo sequence.36, 351, 385 Figure 6.4 Schematic outline of a spin echo sequence. (The Ohio State University. Reproduced with permission.) TR = time of repetition; TE = time of echo; RF = radiofrequency pulse; SEG/PEG/FEG = slice/phase/frequency encoding gradient (applied for spatial encoding of the MR signal); DAQ = Data acquisition. Figure 6.5 Schematic demonstration of a T1-W sequence. (A) Initially, the net vector of all hydrogen protons (fat, soft tissue, fluid) is aligned with the main magnetic field. (B) Immediately after application of a 90° RF pulse all tissues are in the transverse plane but are quickly separated due to differences in realignment with the main magnetic field (T1 relaxation). Fat has a very short T1 relaxation time, fluid has the longest relaxation time, and soft tissues are intermediate. (C) The second 90° RF pulse is applied when fat protons have realigned with the main magnetic field. (D) Fat is now alone in the transverse plane and gives the strongest MR signal, i.e. it appears bright on the resultant image. Fluid has the least transverse magnetization and appears dark; soft tissue is intermediate in its intensity. (The Ohio State University. Reproduced with permission.) Figure 6.6 Transverse pre- (A) and post- (B) T1-W images of the brain of a dog with a large plaque-like meningioma of the left frontal, parietal, and temporal lobes. Fat associated with subcutaneous tissue and bone marrow and contrast-enhancing tissues appear hyperintense, fluid is hypointense, and soft tissues are intermediate. Additional mass effect beyond the margins of the contrast-enhancing lesion is indicated by midline shift and compression of the left lateral ventricle. The underlying reason (vasogenic edema) is not clearly identified using this sequence (see Fig. 6.8 and Fig. 6.10). Figure 6.7 Schematic demonstration of a T2-W sequence. (A) Initially, hydrogen protons in the same tissue are aligned with the main magnetic field and are in phase with each other. (B) Immediately after the 90° RF pulse hydrogen protons in the same substance (fat, soft tissue, fluid) precess in sync with each other in the transverse plane, and all tissues give a strong and uniform signal. (C) Spinning protons quickly lose their coherence due to interference with each other (T2 relaxation). Fluid has the longest T2 relaxation time; fat the shortest. (D) If waiting a long time until listening for the echo (long TE), protons in fat and soft tissues have lost most of their phase coherence. Fluid maintains the strongest transverse magnetization and appears bright on the resultant image. For SE sequences a 180° pulse is applied at TE/2 to cancel out external field inhomogeneities. (The Ohio State University. Reproduced with permission.) Figure 6.8 Transverse T2-W image of the brain of a dog with meningioma (same dog as Fig. 6.6 and Fig. 6.10). Fluid within the ventricular system is strongly hyperintense. The peripheral plaque-like mass is isointense to hyperintense to normal brain parenchyma. Extensive T2 hyperintensity to the white-matter tracts of the left cerebral hemisphere and associated mass effect are consistent with vasogenic brain edema. These sequences are based on conventional SE principles, but additional pulses are applied to selectively suppress signal from certain tissues (inversion recovery sequences) or to accelerate data acquisition (FSE or TSE techniques and single-shot techniques). Figure 6.9 Schematic demonstration of a FLAIR sequence. (A) Initially, the net vector of all hydrogen protons (fat, soft tissue, fluid) is aligned with the main magnetic field. (B) Immediately after application of the initial 180° RF pulse (“inversion pulse”), the vectors of different tissues are flipped out of plane together but are quickly separated due to differences in T1 relaxation. Fat has a very short relaxation time, fluid has the longest relaxation time, and soft tissues are intermediate. (C) The 90° RF pulse is applied when fat has almost realigned with the main magnetic field and fluid has reached the transverse plane. (D) Fluid is now furthest away from the transverse plane and gives off no signal, i.e. it is effectively nulled. (The Ohio State University. Reproduced with permission.) Figure 6.10 Transverse T2-W FLAIR image of the brain of a dog with meningioma (same dog as Fig. 6.6 and Fig. 6.8). Pure fluid within the ventricular system is attenuated, while hyperintensity to the white-matter tracts of the left cerebral hemisphere consistent with vasogenic edema persists. The plaque-like peripheral mass is isointense to adjacent tissues and not clearly seen. Figure 6.11 Schematic demonstration of a STIR sequence. (A) Initially, the net vector of all hydrogen protons (fat, soft tissue, fluid) is aligned with the main magnetic field. (B) Immediately after application of the initial 180° RF pulse (“inversion pulse”), the vectors of different tissues are flipped out of plane together but are quickly separated due to differences in T1 relaxation. Fat has a very short relaxation time, fluid has the longest relaxation time, and soft tissues are intermediate. (C) The 90° RF pulse is applied when fat has reached the transverse plane while fluid and soft tissue lag behind. (D) Fat is now furthest away from the transverse plane and gives off no signal, i.e. it is effectively nulled. (The Ohio State University. Reproduced with permission.) Figure 6.12 Sagittal T2-W FSE (A) and STIR (B) images of the thoracolumbar spine in a dog following trauma. Traumatic intervertebral disc herniation and mild subluxation at T12/13 along with multifocal disc degenerative changes are evident on both sequences. (A) Multifocal T2 hyperintensities within the vertebral bodies (most obvious in the caudal half of L2; arrowhead) are seen. (B) Most vertebral hyperintensities are attenuated (arrowhead), indicating that they are consistent with fatty degeneration of bone marrow. The cranial half of T13 remains hyperintense, consistent with bone contusion. Additionally, extensive subcutaneous edema dorsal to the lumbar spine (*) and patchy hyperintensities in the epaxial muscles dorsal to T12/13 are accentuated due to suppression of adjacent fat. Figure 6.13 Sagittal T2-W FSE (A) and half-Fourier-acquisition single-shot turbo spin echo HASTE (B) images of a dorsal subarachnoid cyst/diverticulum at T13/L1. The lesion is accentuated on the heavily T2-W (myelographic) single-shot sequence. While the generation of SE images relies on the application of pairs of RF pulses, GRE sequences utilize only one initial RF pulse in conjunction with gradient field reversals (Fig. 6.14). GRE sequences use smaller flip angles and shorter TRs than SE sequences (Table 6.2), resulting in shorter scan times. The lack of a 180° pulse has important implications for image weighting and quality: (1) conventional GRE sequences can be used to acquire T1-W, PD-W and T2*-W images—acquisition of truly T2-W images is not possible—and (2) GRE sequences are prone to susceptibility artifacts as there is no compensation for external field inhomogeneities. A plethora of GRE applications have been developed in recent years, including conventional, steady-state, coherent, incoherent (spoiled), steady-state-free precession, balanced, fast, single-shot and echoplanar imaging (EPI) sequences. A comparison between sequences developed by different vendors is difficult as a uniform nomenclature system does not exist. For example, vendor-specific names/acronyms used for a similar coherent gradient echo sequence are “FISP” (Siemens), “GRASS” (GE), “FFE” (Philips), “Rephased SARGE” (Hitachi) and “SSFP” (Toshiba). Rapid development in the field of GRE sequences led to numerous new and advanced applications of MR imaging, such as motion-free (breath hold) abdominal imaging, 3D volumetric imaging, and 3D MR angiography (MRA). Some GRE sequences more or less routinely used for the imaging of the CNS in small animals at this point include T2*-W GRE, T1-W 3D GRE, 2D/3D volumetric acquisition, and magnetic resonance angiography (MRA). Figure 6.14 Schematic outline of a gradient-echo sequence. In contrast to SE techniques only a single RF pulse is applied which is used in conjunction with gradient reversals. (The Ohio State University. Reproduced with permission.) TR = time of repetition; TE = time of echo; SSG/PEG/FEG = slice/phase/frequency encoding gradient (applied for spatial encoding of the MR signal). Table 6.2 Influence of acquisition parameters on image contrast in a gradient echo sequence.37, 385 Figure 6.15 Transverse T2-W FSE (A) and T2*-W GRE (B) images of the brain in a dog with a hemorrhagic infarct. (A) An irregular heterogeneous T2 hyperintense mass is associated with the right frontal lobe. Diffuse T2 hyperintensity extending along the adjacent white-matter tracts is consistent with vasogenic edema. (B) The mass is strongly hypointense on T2*-W image, consistent with susceptibility artifact and indicative of hemorrhage. Figure 6.16 Postcontrast transverse T1-W GRE (volume interpolated breath hold examination; VIBE) image of the brain in a dog with left-sided facial paralysis (slice thickness 0.9 mm). Contrast enhancement of the left facial nerve is noted (arrow), consistent with facial neuritis. Figure 6.17 Ischemic cerebellar infarct in a dog. (A) The transverse T2-W image demonstrates a sharply marginated wedge-shaped hyperintense lesion associated with the left cerebellar hemisphere. (B, C) The lesion remains hyperintense on the diffusion-weighted image (B) and is hypointense on the ADC map (C), consistent with restricted diffusion and ischemic stroke. Figure 6.18 Diffusion tensor imaging of the corticospinal tract in a dog (sagittal view). (Jacqmot et al., 2013. Reproduced with permission from Wiley.)143 Figure 6.19 Functional MRI (fMRI) demonstrating increased activity in the area of the right caudate nucleus (CD) in response to a hand signal (image summed from data acquisition in two awake dogs). (From Berns et al., 2012. Reprinted with permission.)25 Figure 6.20 Transverse postcontrast T1-W SE images of the canine brain without (A) and with (B) fat saturation. (A) No abnormalities are detected. (B) Suppression of adjacent bone marrow fat allows identification of meningeal enhancement, most obvious along the right occipital lobe. A detailed discussion of MR artifacts is beyond the scope of this chapter. However, some important artifacts frequently encountered when imaging the CNS in small animals will briefly be discussed. Figure 6.21 CSF flow artifact. A strongly hyperintense area is observed within the mildly dilated fourth ventricle on transverse T2-W FLAIR image (arrow). This was not identified on other sequences, ruling out a true intraventricular lesion. Figure 6.22 Chemical shift artifact observed at the border of subarachnoid space and epidural fat in the lumbar spine of a dog. The frequency encoding gradient has been applied in a laterolateral direction. The chemical shift artifact results in a black crescent to the left and a white crescent to the right of the spinal cord (arrows) due to misregistration of the signal from fat bordering fluid. This may be confused with a lateralized lesion and is best avoided by applying the frequency encoding gradient in a dorsoventral or ventrodorsal direction. MRI contrast agents most commonly used in veterinary medicine are gadolinium-based. Contrast medium is administered at a dose of 0.1 mmol/kg, which may be increased to improve detection of poorly enhancing lesions. Enhancement is seen if a lesion is vascularized and is located outside the blood–brain barrier. Gadolinium-based contrast media predominantly affect T1 relaxation, and enhancing lesions appear hyperintense on T1-W images (see Figs 6.6, 6.16, 6.20). Certain normal intracranial structures outside of the blood–brain barrier—such as pituitary gland, choroid plexus, trigeminal nerve, and blood vessels—show physiologic contrast uptake. Some disagreement exists as to the optimal timing of MR image acquisition following contrast medium administration (immediate vs. delayed). However, at least for the brain, postcontrast images are usually acquired in more than one plane, and immediate and delayed images are therefore acquired inadvertently. Dynamic studies monitoring contrast enhancement (wash-in and wash-out) over time are not commonly used in veterinary medicine at this point but may be useful for the evaluation of the pituitary gland, cerebral perfusion, and brain tumors. MR contrast agents are considered very safe for use in veterinary patients, and reports of adverse effects are limited to one publication describing suspected anaphylactoid reactions in three dogs. MRI guided tissue sampling is rapidly gaining popularity in human medicine due to continued improvements in the field of MRI-compatible instruments and technologies. While it is not commonplace in veterinary neuroradiology at this point, initial studies have shown promising results. Many disorders of the brain can result in similar MR findings, and some intracranial abnormalities can be detected as incidental findings unrelated to a patient’s clinical presentation. Therefore, familiarity with signalment (species, breed, sex, and age), normal anatomy and variants, and pertinent history (clinical signs, time of onset of clinical signs, course of disease, concurrent or previous diseases) are crucial when evaluating brain MRI scans. Intracranial lesions may be extra-axial (i.e. originating outside actual brain parenchyma) or intra-axial (originating from brain parenchyma). Differential diagnoses for extra-axial lesions include certain inflammatory (e.g. meningitis), neoplastic (e.g. meningioma, nasal tumor), and traumatic lesions (e.g. epidural/subdural hematoma). Differential diagnoses for solitary intra-axial lesions include neoplasia, hematoma, cyst, abscess/granuloma, and infarct. Although inflammatory brain diseases usually manifest as multifocal lesions, solitary masses may be encountered on occasion. Masses in specific locations may allow a presumptive diagnosis of certain tumor types (e.g. pituitary tumor, nerve sheath tumor, medulloblastoma). Differential diagnoses for multifocal brain lesions include metabolic/toxic brain disease, inflammatory brain disease, infarcts, and some intracranial neoplasms (lymphoma, disseminated histiocytic sarcoma, metastases, occasionally meningiomas and other primary brain tumors). A variety of pathologic changes can be associated with various brain diseases, including hydrocephalus, vasogenic edema, mass effect, brain herniation, and hemorrhage. Hemorrhage will be covered under “Acquired brain disorders” later in the chapter. MRI findings in hydrocephalus include dilation of one or more ventricles and/or dilation of the subarachnoid space. In most cases, abnormal CSF accumulation appears hyperintense on T2-W images, hypointense on T1-W images, and attenuates on FLAIR (Fig. 6.23). If CSF contains abnormal cells and/or protein (e.g. in cases of inflammation or intraventricular hemorrhage), altered signal intensity may be observed. Hydrocephalus may be associated with periventricular edema characterized by periventricular T2 hyperintensity, which is most obvious on FLAIR images. In extreme cases CSF may dissect along periventricular white matter, creating diverticula and clefts. Dependent on the etiology of hydrocephalus, potential concurrent findings include other congenital anomalies, an intracranial mass, or signs of trauma. Imaging diagnosis of pathologic hydrocephalus can be challenging. In one study describing ultrasound evaluation of canine ventricles, normal lateral ventricular height was reported to be 0–14% of dorsoventral height of the cerebral hemisphere, moderate ventricular enlargement was defined as 15–25%, and > 25% was considered indicative of severe ventricular enlargement. However, ventriculomegaly and ventricular asymmetry are common findings in asymptomatic animals, and this finding may or may not be clinically significant. Progressive dilation of ventricles and subarachnoid space are also anticipated findings with increasing age and may not be pathologic. Therefore, imaging diagnosis especially of mild ventricular and/or subarachnoid space dilation should be judged in the light of clinical presentation. Figure 6.23 Congenital hydrocephalus in a 2-mo-old Golden Retriever. T2-W transverse image shows severe dilation of the lateral and third ventricles. Figure 6.24 T2-W sagittal image of the brain of a dog with severe diffuse brain disease and swelling. There is subtentorial herniation of the occipital lobes and herniation of the cerebellum through the foramen magnum. Figure 6.25 Transverse T2-W FLAIR image of the brain in a young dog with seizures and presumptive seizure-induced brain changes. There is hyperintensity to the piriform lobes, more severe on the right. Congenital hydrocephalus is most commonly seen in toy and brachycephalic breed dogs and appears as dilation of the ventricular system of variable severity as described above (see Fig. 6.23). Hydranencephaly manifests as a near complete destruction and/or lack of development of the neocortex and has been described in two kittens following intrauterine parvovirus infection. Affected animals show a reduction of size of one or both cerebral cortices to a thin mantle surrounding a large, centrally located cavity. Porencephaly appears as cystic cavities in the cerebrum due to cell destruction or failure of development. These cavities show signal typical of CSF on MRI and may communicate with ventricles or subarachnoid space (Fig. 6.26). Figure 6.26 Porencephaly in an 8-yr-old pug. There is a large CSF isointense lesion associated with the left cerebral hemisphere which is confluent with the left lateral ventricle and the subarachnoid space. This was an incidental finding when an MR examination of the cervical spine was performed. Lissencephaly is a disorder of cortical neuronal migration characterized by paucity, absence, and/or hypoplasia of cerebral gyri and thickening of the cerebral cortex. The disease has been reported in dogs and cats and appears to be hereditary in Lhasa Apsos. MRI findings include a smooth cerebral surface and a thick neocortex with absence of the corona radiata. Holoprosencephaly (HPE) is a failure of the forebrain to bifurcate normally and is characterized by an absence or reduction in the size of midline prosencephalic structures (corpus callosum, septum pellucidum, septal nuclei, fornix, and optic nerves), incomplete separation of normally paired forebrain structures (lateral ventricles, cingulate gyri, and caudate nuclei), and hydrocephalus. Dependent on severity, HPE can be subdivided into alobar, semilobar, and lobar HPE. Agenesis or dysgenesis of the corpus callosum is a feature of HPE but may also occur as an isolated abnormality. Miniature Schnauzers appear to be predisposed both for this condition as well as for lobar HPE. Agenesis or dysgenesis of the corpus callosum is best seen in midsagittal MR images. On transverse images, unusually upturned, pointed corners of the lateral ventricles are evident (Fig. 6.27). Figure 6.27 Dysgenesis of the corpus callosum in a 4-mo-old Miniature Schnauzer presented with hypodipsic hypernatremia. The corpus callosum is small, and the lateral ventricles have unusually upturned and pointed corners. Protrusion of meninges alone or meninges along with brain tissue through a calvarial defect are termed meningocele and meningoencephalocele, respectively. Superficial meningoencephaloceles may be diagnosed clinically; however, diagnosis of basal or ethmoidal meningoencephaloceles requires advanced imaging. Ethmoidal encephaloceles are characterized by a defect in the cribriform plate with rostral herniation of the olfactory bulb(s) (Fig. 6.28). Figure 6.28 Ethmoidal encephalocele in a 10-mo-old dog. The dorsal T2-W image demonstrates absence of the left portion of the cribriform plate and herniation of the left and part of the right olfactory bulb into the caudal nasal cavity. Rathke cleft cysts are pituitary cysts containing mucoid or, less commonly, serous fluid and cellular debris and appear as cystic lesions in the middle cranial fossa. They are hypointense on T1-W images, hyperintense on T2-W images, may show mild ring enhancement, and may not suppress on FLAIR due to composition of fluid. Chiari malformations are a group of structural defects involving brain stem, cerebellum, upper spinal cord, and surrounding bony structures in humans. Secondary formation of a cystic cavity within the cervical spinal cord parenchyma and/or dilation of the central canal (syringomyelia) are common. A disorder similar to Chiari type I malformation in humans termed “Chiari-like malformation and syringomyelia” has been reported in dogs. Cavalier King Charles Spaniels are most commonly affected, but the disease is seen in a variety of breeds and can be found in symptomatic and asymptomatic animals. The condition is characterized by crowding of the caudal fossa resulting in attenuation of the subarachnoid space surrounding the cerebellum, compression and, in severe cases, herniation of the cerebellum into or through the foramen magnum, which is best demonstrated on T2-W median-sagittal images. Additional findings include a focal bending of the cranial aspect of the spinal cord, hydrocephalus, and syringomyelia (Fig. 6.29). Figure 6.29 Chiari-like malformation in a 3-yr-old Cavalier King Charles Spaniel. Sagittal T2-W image of brain and cranial cervical spine shows crowding of the caudal fossa with compression of the cerebellum, mild generalized ventriculomegaly, and linear to tubular T2 hyperintensity associated with the central and dorsal aspect of the cervical spinal cord, consistent with syringomyelia. Atlanto-occipital overlapping without prior trauma is a recently recognized anomaly in small- and toy-breed dogs, which is usually associated with other abnormalities such as Chiari-like malformation, syringomyelia, occipital dysplasia, or atlantoaxial instability. The abnormality is best identified on midsagittal images, which reveal craniodorsal displacement of the atlas and overlap of its lamina with the occipital bone (Fig. 6.30). Figure 6.30 Atlanto-occipital overlapping in a 1-yr-old Yorkshire Terrier. There is abnormal angulation and cranial displacement of the atlas into the cranial vault with marked cerebellar compression. Mild syringomyelia of the cranial cervical spinal cord is also noted. Cerebellar hypoplasia can occur as a primary developmental defect or secondary to viral infection in utero, most commonly parvovirus. The cerebellum may appear small, with increased CSF signal noted around and extending into the folia. Congenital cerebellar abnormalities may not always be apparent on MRI examination; and no abnormalities were detected in Coton de Tuléar dogs with neonatal cerebellar ataxia. Differentiation of true cerebellar hypoplasia from degenerative disease (cerebellar atrophy, abiotrophy, degeneration; see below) may not be possible solely based on imaging findings. The Dandy–Walker malformation complex in human patients refers to a group of congenital CNS anomalies that primarily involve the cerebellum and adjacent tissues. The primary abnormality is partial or complete absence of the cerebellar vermis and cystic dilation of the fourth ventricle. Comparable cases of cerebellar vermian aplasia or hypoplasia and associated cystic dilation of the fourth ventricle have been reported in dogs. Possible concurrent findings include generalized ventricular enlargement, extension of the cystic fourth ventricle into the supratentorial space with displacement of the occipital lobes, reduced size of the cerebellar hemispheres, widening and irregular gyrification of cerebral sulci, and absence of the corpus callosum. Polymicrogyria is a disorder of cerebrocortical development resulting in an increased number of small, disorganized gyri in the dorsal and lateral cerebral cortex. It has been reported in Standard Poodles in which a hereditary basis is suspected. On MRI abnormal gyri are best seen on dorsal T2-W images. Concurrent ventriculomegaly is common. Intracranial epidermoid and dermoid cysts are benign space-occupying lesions that originate from remnants of ectodermal tissue due to defects of neural tube closure. They are often located in the cerebellopontine angle or the fourth ventricle. Signal intensity is variable and dependent on cyst content: cysts with a high lipid content appear hyperintense on T1-W and T2-W images, while cysts with lower lipid content appear hypointense on T1-W images and hyperintense on T2-W images. Dermoid cysts containing adnexa (e.g. hair) may show suspended low-intensity foci in all sequences. Cysts often contain protein and keratin and therefore do not attenuate on FLAIR (Fig. 6.31). They usually do not show contrast enhancement, although ring enhancement may occasionally be noted. Figure 6.31 Epidermoid cyst in a dog. A large mostly cystic mass with a ventral solid component is associated with the fourth ventricle. Incomplete suppression of the cystic component on this FLAIR image is attributed to the protein content of the fluid. Intracranial intra-arachnoid cysts (more appropriately termed diverticula) arise from splitting/duplication of the arachnoidea and occur in close association with an intracranial arachnoid cistern. They are usually considered a primary malformation, although they may develop secondary to inflammation or trauma. Quadrigeminal cistern cysts dorsal to the quadrigeminal plate are most common, but cerebellomedullary cistern cysts have also been reported. Intracranial intra-arachnoid cysts contain fluid isointense to CSF, with attenuation on FLAIR and no evidence of contrast enhancement (Fig. 6.32). Hemorrhage into intracranial intra-arachnoid cysts may change the signal intensity. Quadrigeminal cysts are of variable significance and are frequently incidental. One study found that compression of the occipital lobe by the cyst greater than 14% on median-sagittal image was always associated with clinical signs, while no association was found between the degree of cerebellar compression and clinical signs. Figure 6.32 Incidental quadrigeminal cistern cyst in a 10-yr-old Chihuahua presented with cervical intervertebral disc disease. The sagittal T2-W image shows a strongly hyperintense smoothly marginated area extending caudodorsally from the mesencephalon which results in compression of the cerebellum and to a lesser degree the occipital lobes. Other cystic lesions documented in the caudal fossa in dogs include cerebellar ependymal cysts and choroid plexus cysts. On MRI these are well circumscribed, strongly hyperintense on T2-W, and of variable intensity on FLAIR images. After contrast medium administration they may be nonenhancing, ring-enhancing, or, surprisingly reported in a case of a choroid plexus cyst, strongly enhancing. Inflammatory brain diseases can affect brain parenchyma (encephalitis), meninges (meningitis), or both (meningoencephalitis). Dependent on underlying etiology, involvement of the spinal cord (myelitis/meningomyelitis) may occur. Encephalitis may cause no detectable abnormalities on MRI or may manifest as multifocal (rather than focal) lesions associated with brain parenchyma which typically appear hyperintense on T2-W images and hypointense on T1-W images. FLAIR has higher sensitivity than conventional SE sequences in detecting subtle brain lesions in dogs with clinical signs of multifocal brain disease, and its use is encouraged in all of these cases. Meningitis may not be detected with MRI or may appear as meningeal enhancement following administration of contrast medium (see Fig. 6.20). Canine distemper virus (CDV) and feline coronavirus (FCoV; previously called “feline infectious peritonitis virus,” FIPV) are the most common causes of viral encephalitis in dogs and cats, respectively. In acute CDV infection, T2 hyperintense lesions and loss of contrast between gray and white matter on T2-W images may be found in the cerebellum and/or brain stem, corresponding to areas of demyelination. T2 hyperintense areas are occasionally seen in the temporal lobes, which may be related to infection or postictal edema. MRI findings in chronic distemper meningoencephalitis include essentially bilaterally symmetric T2 hyperintensity of the cortical gray/white matter junction of the parietal and frontal lobes, T2 hyperintensity of the arbor vitae of the cerebellum with partial loss of cerebellar cortical gray/white matter demarcation, subtle focal T2 hyperintensity of the pons, and meningeal contrast enhancement. In cats with feline infectious peritonitis (FIP) affecting the central nervous system MRI may show T2 hyperintensity and contrast enhancement of the ventricular lining, choroid plexus, and meninges compatible with ependymitis, choroiditis, and meningitis (Fig. 6.33). Concurrent findings may include hydrocephalus, syringomyelia, and herniation of the cerebellum secondary to increased intracranial pressure. A study investigating MRI findings in dogs experimentally infected with rabies found parenchymal abnormalities affecting the hippocampus, hypothalamus, basal ganglia, brain stem, and white matter which were more pronounced in the paralytic than the furious stage of the disease. Figure 6.33 Dorsal postcontrast T1-W image with fat saturation of a 7-mo-old Siamese cat with presumptive diagnosis of FIP. There is mild hydrocephalus and contrast enhancement along the ventricular lining, the choroid plexus and to a lesser degree the meninges, consistent with ependymitis, choroiditis, and meningitis. Mechanisms of bacterial infection of the CNS in cats and dogs include hematogenous spread, contiguous infection from adjacent structures (middle/inner ear, nasal cavity, sinuses, orbit, skull, vertebrae), direct inoculation (trauma, bite wound, surgery), and migration of foreign bodies or aberrant parasites. In addition to meningitis, diffuse cerebritis, and meningoencephalomyelitis, CNS infection may result in focal parenchymal abscesses or empyema in the subdural or epidural space. MRI features of intracranial infection secondary to plant foreign body migration, hematogenous spread from a mediastinal abscess, bacterial endocarditis, local extension from orbital disease and from osteomyelitis of the sphenoid bone, and as complication of otitis media or interna have been described in small animals. Intracranial abscesses are typically hypointense on T1-W and hyperintense on T2-W images, with strong peripheral contrast enhancement and associated brain edema (Fig. 6.34). Concurrent meningitis appearing as meningeal enhancement and/or thickening is common. Dependent on lesion location and extent, additional findings might include hydrocephalus and/or brain herniation. Neurotuberculosis (tuberculous meningitis, cerebral tuberculoma) results from hematogenous spread of organisms of the Mycoplasma tuberculosis complex and is infrequently reported in animals. MRI findings in one affected dog included a large heterogeneous T2 isointense to hypointense extra-axial mass with patchy peripheral contrast enhancement and hyperostosis and erosion of adjacent skull bones. Figure 6.34 Brain abscess in a 2-yr-old dog several weeks after a bite wound to the head. The transverse postcontrast T1-W GRE image with fat suppression demonstrates a rounded strongly ring-enhancing mass with a nonenhancing center in the right frontal lobe. Additional finding include soft-tissue irregularities and a defect in the overlying skull, and hypointensity and mass effect to the entire right cerebral hemisphere consistent with edema. Fungal infections (Cryptococcosis, Phaeohyphomycosis, Aspergillosis, Blastomycosis, Histoplasmosis) have been reported to affect the CNS in dogs and cats. MRI findings are variable and may include intra-axial or extra-axial masses of variable contrast enhancement, gelatinous pseudocysts, meningeal enhancement, enhancement of the ventricular lining, and intra-cranial extension of nasal or retro-orbital masses (Fig. 6.35). Associated findings may include edema, hydrocephalus, syringohydromyelia, and brain herniation. Figure 6.35 Blastomycosis in a cat. Dorsal T1-W postcontrast T1-W GRE image with fat suppression (reconstructed from transverse dataset) shows severe panophthalmitis of the right eye and two strongly contrast-enhancing intracranial masses. Protothecosis is a rare infection with the achlorophyllic algae Prototheca, and only a few reports of CNS involvement are found in the veterinary literature. MRI findings in one dog included multifocal T2 hyperintense intra-axial lesions, ventriculomegaly, cerebellar herniation, syringomyelia, and meningeal enhancement. Parasitic meningoencephalitis in dogs and cats is caused by aberrant migration of parasites such as Dirofilaria, Baylisascaris, Cuterebra, Taenia, Ancylostoma, Toxascaris, and Angiostronglylus. MRI features include focal or multifocal parenchymal lesions of variable signal intensity and peripheral parenchymal and/or meningeal contrast enhancement. Intraparenchymal hemorrhage is a common feature in parasite migration, and T2*-W images are especially useful in establishing a (presumptive) diagnosis. Protozoal meningoencephalitis may be caused by a variety of organisms. MRI features in dogs infected with Neospora include cerebellar atrophy with T2 hyperintense material surrounding the cerebellum and extending into the sulci, loss of contrast between cerebellar gray and white matter, T2/FLAIR hyperintensities within the cerebellum, and meningeal contrast enhancement. In two dogs with systemic Leishmaniasis nonenhancing T2 and FLAIR hyperintense lesions in thalamus and brain stem found on MRI were attributed to ischemic infarcts secondary to systemic necrotizing vasculitis. Toxoplasma infection in cats may manifest as multifocal indistinct T2 hyperintense contrast-enhancing parenchymal lesions with associated brain edema. Several inflammatory conditions unrelated to infectious agents have been identified in dogs which generally respond to immunosuppressive treatment suggesting immune mediated etiology. Attempts have been made to separate these into specific diseases based on breed (e.g. “Pug dog encephalitis”) and other criteria, however, due to overlap in clinical, diagnostic and pathologic findings a definitive premortem diagnosis is difficult. These disorders may be summarized under Meningoencephalomyelitis of undetermined etiology (MUE). Granulomatous meningoencephalitis (GME) can affect any breed of dog but most often occurs in young to middle-aged toy breeds. The disease can affect the brain and/or spinal cord. On MRI, GME lesions can be focal or multifocal and commonly affect the brain stem. Although the disease has a predilection for white matter, it is not associated with distinct topography. Lesions are typically hyperintense on T2-W and FLAIR images, iso- to hypointense on T1-W images and variably contrast enhancing (Fig. 6.36). Meningeal enhancement may or may not be observed. In ocular GME, MRI may show enlargement of the optic chiasm and fairly symmetric contrast enhancement of the optic nerves, optic chiasm, and visual pathways. Figure 6.36 MUE (GME, presumptive) in a 4-yr-old Maltese. The T2-W sagittal image demonstrates an extensive diffuse hyperintense lesion associated with the brainstem. Necrotizing meningoencephalitis (NME) is characterized by cavitary necrosis in the neuroparenchyma. The disease was initially described in the pug breed (“pug dog encephalitis”), but similar disorders have since been reported in other small breeds including Maltese, Chihuahua, Pekingese, French Bulldog, Shi Tzu, and Lhasa Apso. A distinct form of NME described mainly in Yorkshire Terriers has been termed necrotizing leukoencephalitis (NLE). Descriptions of imaging findings in this group of inflammatory brain disorders are not available for all breeds. NME in pugs is usually limited to the forebrain. MRI findings include diffuse asymmetric cerebral lesions which are nonuniformly hyperintense on T2-W images, isointense to hypointense on T1-W images, and affect gray and white matter resulting in loss of gray/white matter distinction. Additional findings include variable degrees of contrast enhancement of brain and leptomeninges, enlarged and asymmetric lateral ventricles, mass effect, brain herniation, and T2/FLAIR hyperintensity associated with the hippocampus and piriform lobes. MRI findings reported in Chihuahuas and French Bulldogs with NME are similar; however, brain stem involvement seems more common. In almost all cases of NLE reported in Yorkshire Terriers, lesions are located in the cerebrum and brain stem and appear iso- to hypointense on T1-W images, hyperintense on T2-W and FLAIR images, and show variable contrast enhancement. Concurrent hydrocephalus of variable severity is possible. Greyhound nonsuppurative meningoencephalitis manifests as T2/FLAIR hyperintense lesions with minimal or absent contrast enhancement associated with olfactory lobes, frontal and frontotemporal gray matter, and caudate nuclei bilaterally. MRI in dogs with idiopathic eosinophilic meningitis/meningoencephalitis may be normal or show patchy regions of T2 hyperintensity and contrast enhancement in the cerebral cortex, solitary or multiple masses, meningeal enhancement, or enlargement and contrast enhancement of cranial nerves. In a boxer with cerebral extension of steroid-responsive meningitis arteritis, MRI findings included symmetrical multifocal to diffuse T2/FLAIR hyperintense changes of the cerebral gray matter and ependymal lining, increased periventricular signal, and a large amount of intraventricular sediment. A case of lymphocytic meningoencephalitis in a cat was characterized by multifocal T1 isointense and T2 hyperintense contrast-enhancing lesions. No intracranial abnormalities were detected on MRI in a cat diagnosed with histiocytic encephalitis. Trigeminal neuritis in dogs is characterized by diffuse enlargement of the nerves and is usually bilateral. Affected nerves are isointense to brain parenchyma on T1-W and PD-W images, isointense or hyperintense on T2-W images and show contrast enhancement. The term “cerebrovascular diseases” refers to all disorders in which there is an area of brain transiently or permanently affected by ischemia or bleeding and/or in which one or more blood vessels of the brain are primarily impaired by a pathological process. An aneurysm is an abnormal focal enlargement of an artery of variable etiology. Patent aneurysms appear as signal void on both T1-W and T2-W images representing fast-flowing blood. Small aneurysms and aneurysms with turbulent flow are unreliably shown on conventional MRI and are better demonstrated by magnetic resonance angiography (MRA). While fairly common in humans, intracranial aneurysms are rarely diagnosed in veterinary patients. A case of a presumed aneurysm, most likely the result of traumatic arteriovenous fistulization, has been described in a dog. On CT images, an expansile enhancing mass was present along the intracranial cavernous sinus and extended through the orbital fissure into the retrobulbar space. With MRI, the structure appeared as a signal void due to the presence of rapidly flowing blood. The vascular origin of the lesion was confirmed with MRA. Cerebrovascular malformations are congenital anomalies of brain vasculature. Different types include arteriovenous malformations (clusters of abnormal arteries and veins with direct arterial-to-venous shunts), venous malformations (anomalous veins separated by normal neural parenchyma), cavernous malformations (masses of contiguous sinusoidal vessels with no intervening parenchyma), and telangiectasias (masses of small capillary-type vessels separated by normal parenchyma). Animals with cerebrovascular malformations usually present with intracranial hemorrhage, and imaging findings are consistent with hemorrhagic stroke (see below). Identification of associated large vessels suggests vascular malformation as the underlying etiology of spontaneous intracranial hemorrhage. However, compression or obliteration of vessels by hematoma, extremely slow flow, and thrombosis may obscure the abnormal vessels, and specialized techniques (catheter angiography, repeat MRI, or MRA) may be needed to achieve a diagnosis. A stroke is a suddenly developing focal neurologic deficit resulting from an intracranial vascular event. In ischemic stroke, blood flow to an area of tissue is compromised due to intracranial arterial or venous obstruction. Hemorrhagic stroke results from the rupture of intracranial blood vessels. Ischemic strokes can be categorized according to anatomic site, size, age, type (pallid or hemorrhagic), pathology (arterial vs. venous), mechanism (thrombotic, embolic, hemodynamic), and etiology. Causes of ischemic stroke in dogs include primary hypothyroidism, hypertension and diabetes, embolic metastatic tumor cells, necrotizing vasculitis due to systemic leishmaniasis, chronic renal disease, hyperadrenocorticism, intravascular lymphoma, septic thromboemboli, fibrocartilaginous embolism, migrating parasite or parasitic emboli (Dirofilaria immitis), and hypercoagulable state associated with hyperadrenocorticism, protein-losing nephropathy (PLN), or neoplasia. In approximately 50% of dogs with ischemic stroke, no underlying condition is identified. In cats, hypertrophic cardiomyopathy, neoplasia (lymphoma), and liver disease including hepatic lipidosis have been identified as potentially predisposing factors. Territorial infarcts occur when one of the main arteries supplying the brain is occluded. Lacunar infarcts are subcortical infarcts limited to the vascular territory of an intraparenchymal superficial or deep perforating artery. Small-breed dogs are more likely to have territorial cerebellar infarcts, and large-breed dogs are more likely to have lacunar thalamic or midbrain infarcts. Cavalier King Charles Spaniels and Greyhounds may be predisposed to infarcts. Watershed infarcts are infarcts in the boundary zone between large artery territories and are uncommon in veterinary patients. Diffusion weighted imaging (DWI) is sensitive to alterations in brain parenchyma following stroke and is capable of demonstrating abnormalities within minutes of an event (see above). Restricted diffusion (impairment of normal Brownian motion) occurs secondary to failure of the cell membrane ion pump and subsequent cytotoxic edema. This appears as marked hyperintensity on DWI and hypointensity on a synthesized ADC map derived from two or more DWI (see Fig. 6.17
Principles and Application of Magnetic Resonance Imaging (MRI)
Magnetic resonance imaging (MRI) of the brain
Silke Hecht
Basic MRI physics135, 142, 235, 278, 351, 354, 385
MR sequences
Basic spin echo (SE) sequences115, 179, 274, 279, 285–287, 293, 385
TR
TE
Weighting
Short (300–700ms)
Short (5–30ms)
T1-W
Long (2000–4000ms)
Long (60–150ms)
T2-W
Long (> 2000–4000ms)
Short (5–30ms)
PD-W
Modified spin echo (SE) sequences23, 50, 83, 94, 95, 131, 179, 217, 269, 293, 309
Gradient recalled echo (GRE)36, 81, 94, 104, 110, 131, 179, 208, 224, 259, 265, 279, 286, 289, 294, 319, 350, 352, 366, 383–385, 387
TR
TE
Flip angle
Weighting
Short (< 50ms)
Short (< 5ms)
Large (70–90°)
T1-W
Long (> 100ms)
Long (15–25ms)
Small (5–20°)
T2*-W
Long (> 100ms)
Short (< 5ms)
Small (5–30°)
PD-W
Functional imaging18, 25, 37, 45, 48, 62, 126, 142, 143, 154, 194, 223, 238, 253, 268, 284, 297, 334, 352, 370, 381, 385, 399
Technical modifications34, 56, 64, 65, 70, 99, 370, 373
Artifacts37, 57, 100, 101, 132, 307, 406
Contrast media in MRI62, 64, 116, 119, 150, 186, 271, 275, 276, 310, 405
MRI guided tissue sampling49, 91, 123
MRI of the brain
MR imaging techniques94, 115, 131, 238, 286, 287
Approach to the MR examination of the brain15, 45, 50, 57, 121, 131, 140, 178, 182, 195, 280, 287, 288, 301, 344, 367, 394
Associated findings in intracranial disease12, 14, 15, 46, 68, 71, 72, 75, 82, 86, 129, 162, 163, 165, 180, 219, 229, 236, 304, 323, 333, 348, 351, 353, 371, 376, 380, 400
Congenital brain disorders
Forebrain (telencephalon and diencephalon)66, 127, 145, 146, 158, 193, 212, 226, 229, 295, 303, 313, 314, 332, 333, 343
Midbrain and hindbrain (mesencephalon, metencephalon, myelencephalon)33, 41, 43, 44, 53, 55, 60, 63, 67, 73, 76, 78, 105, 152, 164, 166, 167, 198, 209, 210, 212, 213, 220, 229, 230, 240, 264, 267, 272, 282, 291, 292, 299, 302, 313, 327, 333, 339, 361, 363, 368, 369, 401
Acquired brain disorders
Inflammatory brain diseases27, 52, 64, 190, 237, 250, 344
Infectious inflammatory brain diseases4, 13, 17, 19–21, 24, 29, 38, 59, 69, 71, 93, 97, 106, 114, 120, 133, 134, 148, 171, 174, 175, 191, 192, 199, 221, 228, 239, 249, 250, 255, 258, 308, 320, 328, 331, 335, 344, 384, 392, 404
Noninfectious inflammatory brain diseases/ meningoencephalomyelitis of undetermined etiology (MUE)30, 40, 51, 58, 89, 137, 138, 151, 155, 157, 168, 172, 188, 189, 204, 206, 298, 326, 356, 375, 396, 402
Other inflammatory intracranial diseases250, 306, 341, 392, 398
Cerebrovascular disease
Intracranial aneurysms and cerebrovascular malformations234, 259, 340, 344, 346, 353
Stroke (cerebrovascular accident)3, 5, 7, 11, 26, 28, 35, 39, 45, 62, 79, 104, 107–109, 112, 114, 117, 126, 141, 148, 149, 153, 160, 181, 182, 196, 208, 216, 232, 263, 300, 329, 333, 345, 347, 352, 354, 383, 384, 389
Stay updated, free articles. Join our Telegram channel
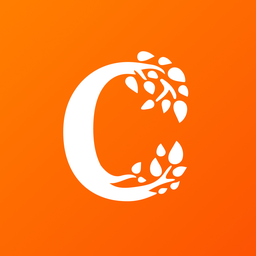
Full access? Get Clinical Tree
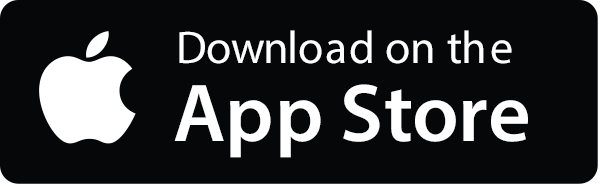
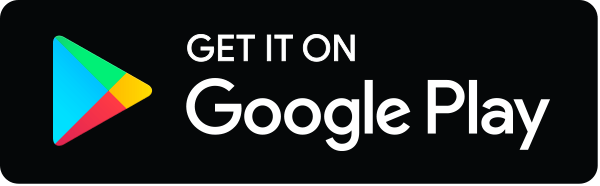