Tiina E. Kähkönen1, Jussi M. Halleen1, and Jenni Bernoulli2 1OncoBone Ltd, Oulu, Finland 2University of Turku, Institute of Biomedicine, Turku, Finland Metastasis refers to spreading of cancer to distant sites of the body (Fidler and Kripke 2015). In the metastatic process, cancer cells migrate from their primary location through the blood or lymph system in other parts of the body and form new metastatic tumors. Metastases are the main cause of mortality in cancer patients, and despite the recent progress in cancer drug development, no therapies are currently available for curing metastasized cancers (Hiraga 2019; Byrne et al. 2019). Our focus in this chapter is in bone, which is a major site of metastasis in bladder, breast, kidney, lung, prostate, thyroid and uterine cancers, and in melanoma (Byrne et al. 2019). Breast and prostate cancer patients are most affected by bone metastases that are developed in 70–85% of patients in advanced stages (Sowder and Johnson 2019). In addition, active bone disease is characteristic to multiple myeloma (MM), and osteosarcoma is a primary bone tumor. The five‐year survival rates dramatically decrease in patients with advanced stages (stage III–IV in breast cancer) where the patients typically have higher number of metastases (Figure 6.1). The advanced stages with metastatic disease can be modeled also in preclinical settings. Success rates in clinical trials are clearly lower in oncology than in any other indication area. During 2000–2015, the success rate was 3.4% in oncology clinical trials and 20.8% in clinical trials of other indications (Wong et al. 2019). One important reason for the poor success rate in oncology may be the use of preclinical efficacy models with poor clinical predictivity. Although it is well known that cancer deaths are caused by metastases, the efficacy of new therapies is usually not tested in preclinical models in metastatic microenvironments. This is of great concern because metastatic microenvironment may dramatically change tumor properties and induce drug resistance. Figure 6.1 Five‐year survival rate in breast cancer patients with different stages of disease, and in vivo images of modeling advanced stages in preclinical settings. In upper image, stage 0 refers to tumors that are noninvasive and localized in the breast. In stage I, lymph node metastases may be present. Stage II or III patients already have metastases in lymph nodes or may have small, not yet clinically detectable metastases in distant sites, including bones. Stage IV patients have a metastatic disease, including metastases in the skeleton. As the disease progresses from stage 0 to IV, the five‐year survival rate is dramatically decreased, being about 30% in stage IV patients. The lower image refers to how the disease develops in preclinical breast cancer models, and how the metastases form and grow in different locations, mostly in the skeleton. One good example of the problem is angiogenesis inhibitors that showed good efficacy in subcutaneous models, but no efficacy was observed in the first clinical trials. Retrospective testing in metastasis models showed no efficacy (Kerbel 2015). Angiogenesis is organ‐specific, and in some metastatic locations such as lungs or pancreas the tumors use the existing vasculature instead of neoangiogenesis, the process which would have been blocked by these compounds. Thus, angiogenesis inhibitors did not help patients with lung metastases in clinical setting, which would have been observed in preclinical metastasis models. Oncology drug development community is currently highly focused in developing immunotherapies, and evidence of immunotherapy efficacy on bone metastases is scarce (Almeida et al. 2017; Kähkönen et al. 2021). Such information would be important not only for efficacy but also for safety aspects, knowing the many interactions between bone cells and immune cells that are described in more detail later in this chapter. Bone consists of a strong, dense, and tough outer layer called cortical bone, and a spongy inner layer called trabecular bone. Cortical bone is covered by a periosteum on its outer surface, and an endosteum on its inner surface. Trabecular bone compartment is highly vascular and contains red bone marrow where hematopoietic stem cells (HSCs) are produced (Langdahl et al. 2016). Bone consists of about 25% of organic matrix, of which 2–5% are cells, 5% water, and 70% inorganic hydroxyapatite, which is a crystalline complex of calcium and phosphate. Of bone cells, bone‐forming osteoblasts are derived from mesenchymal stem cells (MSCs) and bone‐resorbing osteoclasts from HSCs. Calcified bone matrix includes osteocytes, cells derived from osteoblasts that have many important functions. Bone modeling refers to an uncoupled process of bone resorption and formation that leads to bone growth and changes in skeletal form during childhood and adolescence, while bone remodeling refers to a coupled event of bone resorption and formation that is constantly ongoing to repair microfractures in the skeleton (Langdahl et al. 2016). Bone turnover is the sum of bone resorption and bone formation processes that occur during bone modeling and remodeling. Uncoupling of bone resorption over bone formation leads to osteoporosis and uncoupling of bone formation over bone resorption leads to osteopetrosis. In oncology, such uncoupling can occur for example through the action of cancer cells in bone metastases (cancer‐induced bone disease, CIBD) or cancer therapies such as hormone deprivation or chemotherapy (cancer treatment‐induced bone loss (CTIBL) (D’Oronzo et al. 2015). The balance of bone resorption and formation is regulated through the receptor activator of nuclear factor kappa‐Β‐ligand (RANKL)/receptor activator of nuclear factor kappa‐Β (RANK)/osteoprotegerin (OPG) system (Yasuda et al. 1998), where RANK in the surface of osteoclast precursor cells is bound to RANKL in the surface of osteoblasts, leading to fusion of the osteoclast precursor cells to form multinuclear osteoclasts. OPG is a soluble decoy receptor that binds RANKL with higher activity than RANK, thereby inhibiting osteoclast formation and protecting bone from excess resorption (Nelson et al. 2012). Bone marrow is primarily considered as a site of hematopoiesis but it is also an important secondary lymphoid organ where immune responses are initiated, maintained and fine‐tuned (Zhao et al. 2012; Pabst 2018; Di Rosa 2009; Stefanovic et al. 2013). Bone marrow contains many immune cell types including T cells, B cells, dendritic cells (DCs), neutrophils, and myeloid‐derived suppressor cells (MDSCs). Of these, T cells are mostly discussed in the following paragraphs. Besides immune cells, bone marrow contains many pro‐ and anti‐inflammatory cytokines such as interferon gamma (IFNγ), tumor necrosis factor alpha (TNFα), many interleukins (ILs), chemokine C‐C motif ligand (CCL) 4, vascular endothelial growth factor (VEGF), epidermal growth factor (EGF), insulin‐like growth factor (IGF), and basic fibroblast growth factor (bFGF) that can modify immune responses (Stefanovic et al. 2013). Immune cells in the bone marrow are important contributors in maintaining health and disease, including cancer. The role of immune cells in bone metastasis is described in the following paragraphs (Figure 6.2). At natural non‐disease state, 3–20% of bone marrow mononuclear cells are lymphocytes distributed in stroma and parenchyma (Zhao et al. 2012; Di Rosa 2009; Stefanovic et al. 2013). Of these, T cells are the second most abundant after MDSCs, and their number in bone marrow is higher than in spleen or lymph nodes (Di Rosa 2009). In mouse bone marrow, 1–5% of mononuclear cells are CD3+ T cells (Zhao et al. 2012; Di Rosa 2009; Stefanovic et al. 2013), of which about 1.5% are CD4+ T cells and 2–2.5% are CD8+ T cells. Over 30% of CD4+ T cells are CD25+ regulatory T cells (Tregs) (Nelson et al. 2012; Pabst 2018; Di Rosa 2009; Stefanovic et al. 2013). CD4+ and CD8+ memory T cells can be recruited to bone marrow from circulation by specific signals. For example, CD8+ T‐cell recruitment can occur via L‐, P‐, and E‐selectins (Di Rosa 2009). Figure 6.2 Overview of osteoimmuno‐oncology (OIO) concept. Three main components regulate bone metastasis: cancer cells, bone cells, and immune cells. When cancer cells grow in bone microenvironment they interact with bone‐resorbing osteoclasts and bone‐forming osteoblasts and their progenitors, HSCs and MSCs, respectively. These interactions lead to either osteolytic (increased bone resorption) or osteosclerotic (increased bone formation) bone metastases, depending on the cancer type. Upon cancer‐induced bone resorption, many growth factors and cytokines are released from the bone matrix, promoting tumor growth and regulating function and activity of many immune cells such as T and B cells, neutrophils, DCs, natural killer cells and MDSCs. The immune microenvironment in bone metastasis is in most cases immunosuppressive, which promotes tumor growth. Immune cells and osteoclasts both originate from the same HSCs, and immune cells, especially T and B cells, have a role in regulating bone turnover through osteoclasts and osteoblasts (Made in ©BioRender – biorender.com). T cells in bone marrow express C‐X‐C chemokine receptor type (CXCR) 4, C‐C chemokine receptor type (CCR) 5, CXCR6, and CXCR31, and they can be activated by inflammatory cytokines such as CCL3, CCL4, CCL5, CXCL16, and CXCL1 (Pabst 2018). Furthermore, antigen‐specific CD8+ memory T cells reside predominantly in the bone marrow and start to proliferate when stimulated by IL‐7 and IL‐15, and their proliferation is inhibited by transforming growth factor beta (TGFβ) (Zhao et al. 2012; Pabst 2018; Di Rosa 2009). Importantly, there are many molecular interactions between T cells and bone marrow cells. Many bone marrow cells such as osteoblasts and osteoclasts express a ligand to which the corresponding receptor‐expressing T cells bind (Zhao et al. 2012). These molecular interactions include for example CXCR4, P‐selectin glycoprotein ligand 1 (PSGL‐1), conjugated linoleic acid (CLA), L‐selectin, α4β7 integrin, very late antigen (VLA) 4 and 5 that contribute to T‐cell trafficking and retention, and IL‐2, 5, and 15 receptors, RANKL and TGF‐β for T‐cell expansion. Natural killer T (NKT) cells represent 0.4–4% of bone marrow cells (Zhao et al. 2012; Stefanovic et al. 2013). NKT cells are located in the bone marrow among other immune organs, and upon activation by antigen‐presenting CD11b+ DCs they start to proliferate in bone (Zhao et al. 2012; Chang et al. 2018; Feuerer et al. 2003). Also, naïve and effector T cells can be found from bone marrow but in lesser extent than in other lymphoid organs (Zhao et al. 2012; Pabst 2018). The number of B cells is about five times lower than the number of T cells in bone marrow (Zhao et al. 2012; Stefanovic et al. 2013). B cells are produced and mature in bone marrow. Osteoblast and CXCL‐12 and IL‐7 expressing cells are important for B‐cell maturation, and the maturation is supported by extrinsic signals coming from the bone marrow (Zhao et al. 2012). Mature B cells secrete‐specific antibodies and are involved in the maintenance of long‐term immunity (Zhao et al. 2012; Pabst 2018; Di Rosa 2009). In mouse bone marrow, 20–30% of all bone marrow cells are MDSCs. Under pathological conditions such as cancer, immature myeloid cells are expanded and activated to become MDSCs, and they can be further divided into granulocytic and monocytic MDSCs (Zhao et al. 2012). These cells are immunosuppressive and produce factors such as arginase I or inducible nitric oxidase synthase or TGF‐β that downregulate immune‐mediated effects on tumors. One to two percent of bone marrow cells are CD11c+ DCs that play a key role in innate and adaptive immune responses (Zhao et al. 2012). Circulating DCs are retained in bone marrow from where they are activated by antigen‐dependent contacts by memory T cells (Zhao et al. 2012). These cells are maintained by CXCL12 and CXCR4 pathways, and upon activation immobilize from the bone marrow and travel to the site of infection (Zhao et al. 2012). About 1% of bone marrow immune cells are antigen‐producing plasma cells (Zhao et al. 2012; Stefanovic et al. 2013) that secrete antibodies and disappear after the immune reaction has been finished (Chang et al. 2018). Osteoimmunology is defined as interactions between immune cells and bone cells. This concept was first introduced in the year 2000, describing T‐cell‐mediated effects on osteoclasts (Arron and Choi 2000). After this, many other interactions between immune and bone cells have been identified, and overall, the link between different cell types has been well established (Di Rosa 2009; Criscitiello et al. 2015; Walsh and Choi 2014). There are many common molecules expressed in both immune and bone cells, and the immune system has an important role in the regulation of bone homeostasis (Di Rosa 2009; Criscitiello et al. 2015). Immune cells and bone resorbing osteoclasts both differentiate from the same CD34+ HSCs, which is the first link between these cell types (Di Rosa 2009; Rauner et al. 2007). When differentiated, osteoclasts and osteoblasts can induce effects on immune cells and vice versa. T cells impact osteoclastogenesis by secreting many cytokines such as IL‐1, IL‐4, IL‐6, and IFNγ (Rauner et al. 2007). By inducing osteoclastogenesis, activated T cells facilitate bone loss in pathological conditions including cancer metastasis to bone (Walsh and Choi 2014). The roles of CD4+ and CD8+ T cells in this process are not clear, but in in vitro conditions, CD8+ T cells have been shown to inhibit osteoclastogenesis (Di Rosa 2009). Also, a T‐cell‐mediated protective effect on bone metabolism has been seen in in vivo studies where T‐ and B‐cell deficient mice have decreased bone mineral density (BMD) (Di Rosa 2009). The role of osteoblasts on immunology has been recently acknowledged. Osteoblasts have antigen‐presenting properties as they express major histocompatibility complex (MHC) II molecules that can activate T cells (Rauner et al. 2007). Also, other immune cells can have an effect on bone, for example macrophages can increase osteoblastogenesis when activated by IL‐18 (Rauner et al. 2007). Besides regulating bone homeostasis, the RANK/RANKL/OPG‐pathway has effects on immune cells and plays a complicated role in regulating immune responses. The RANK/RANKL/OPG pathway is important in lymph node organogenesis and in T‐ and B‐cell development (Criscitiello et al. 2015; Walsh and Choi 2014). RANK signal is mediated by TNF receptor‐activated factors (TRAFs) and of these, TRAF6 has a major role in maturation and activation of both osteoclasts and DCs. RANKL signaling can be regulated by antigen‐specific immune responses and interaction of T cells and DCs. RANKL is expressed on the surface of CD3+ T cells and activated CD4+ and CD8+ cells. RANKL also stimulates survival of DCs and macrophages (Di Rosa 2009; Walsh and Choi 2014). One important relation between the RANK/RANKL/OPG pathway and immune system was revealed in knockout mouse studies, where the RANKL/RANK‐KO mice failed to develop secondary lymphoid organs, including lymph nodes, Peyer’s patches, cryptopatches, and spleen (Walsh and Choi 2014). Also, defects were observed in the development of thymus and T cells. OPG produced by osteoblasts, T cells, B cells, and DCs (Di Rosa 2009) antagonizes RANK/RANKL function and can negatively regulate T‐cell proliferation (Di Rosa 2009; Criscitiello et al. 2015). OPG can also affect DC function and production of cytokines independently of RANK/RANKL signaling (Arron and Choi 2000; Criscitiello et al. 2015). Other proteins important for bone and immune cells include IFN‐y and IL‐4 that inhibit bone resorption, and IL‐17 that stimulates resorption (Di Rosa 2009). Bone‐targeted therapies such as the bisphosphonate zoledronic acid and the human monoclonal anti‐RANKL antibody denosumab are used in bone metastatic patients to inhibit bone resorption, but they may also have effects on immune cells (Criscitiello et al. 2015). Zoledronic acid is the most efficacious from the currently available bisphosphonates on inhibiting osteoclast‐mediated bone resorption in osteolytic metastases, but it also affects δT cells, which partly mediates improved survival of breast cancer and MM patients (Criscitiello et al. 2015). This may happen in two phases, including firstly increased direct T‐cell‐mediated lysis of tumor cells and/or activation of DCs, and secondly indirectly by increasing expression of IPP/ApppI in breast cancer cells, which increases T‐cell‐mediated killing of tumor cells (Stefanovic et al. 2013). Denosumab has been hypothesized to affect T‐, B‐, and DC‐cell survival via the RANK/RANKL/OPG pathway (Criscitiello et al. 2015). Immunomodulation of bone targeting compounds could also be mediated through decreasing monocyte and DC survival and antigen presentation, by disturbing CD4+ T‐cell activation, and by affecting B‐cell production and development (Stefanovic et al. 2013). As seen from the previous chapters, bone and immune system are tightly linked. This raises a question what are the effects of immunotherapies on healthy bone? For example, CTLA‐4 is expressed in activated T cells, and CTLA‐4 knock‐out mice have hyperactive T and B cells and develop lymphoproliferative disorders. Importantly, these mice have increased osteoclastogenesis causing osteopetrosis (Nagahama et al. 2004). Moreover, PD‐1 is expressed also in T and B cells and in myeloid cells. PD‐1 knock‐out mice also exhibit bone defects and have increased trabecular and cortical BMD and bone volume, correlating with decreased number of osteoclasts. Also, other therapies may have unwanted effects on bone via immune cells. For example, activated T cells produce RANKL and cytokines that can be altered by treatment with denosumab or other bone‐targeted agents. Immunotherapy‐related safety concerns are also observed in patients. A case series revealed for the first time immune‐related adverse events (irAEs) in the skeleton (Moseley et al. 2018), including vertebral compression, multiple fractures and resorptive bone lesions. These cases highlight the need to monitor skeletal health in patients that are receiving immunotherapies. The vicious cycle refers to a self‐amplifying process where factors secreted by tumor cells promote bone resorption, leading to the release of factors from bone matrix that further increase tumor growth. In this process, tumor cells can primarily induce bone resorption (osteolytic metastases) or formation (sclerotic/osteoblastic metastases). The classical pathway in osteolytic bone metastases is the parathyroid hormone‐related peptide (PTHrP)‐TGFβ pathway (Guise et al. 1996), where PTHrP secreted by tumor cells induces osteoblasts to stimulate osteoclastic bone resorption through the RANKL/RANK/OPG pathway, leading to increased release of TGFβ and other factors from bone matrix that stimulate tumor cell proliferation, completing the vicious cycle. TGFβ is also important in several cellular functions, such as epithelial‐to‐mesenchymal transition, invasion, angiogenesis, and immune tolerance. Immune cells also play an important role in the vicious cycle. For example, activated T cells express RANKL and are able to induce differentiation of osteoclasts in vitro. TGFβ in turn inhibits the proliferation of T cells, potentially inducing local immunosuppression (D’Amico and Roato 2015). Some cancer cells secrete factors such as endothelin 1 (ET‐1), Wingless and Int1 proteins (Wnts), bone morphogenetic proteins (BMPs), TGFs, fibroblast growth factors (FGFs) and platelet‐derived growth factors (PDGFs) that increase osteoblast differentiation, leading to sclerotic metastases. Also, prostate‐specific antigen (PSA) in prostate cancer cells may have an impact on the formation of sclerotic metastases (Cumming et al. 2011). Prostate cancer cells are also capable of osteomimicry, which means that they can function as osteoblasts and form mineralized bone nodules (Koeneman et al. 1999; Lin et al. 2001). It remains unclear what osteoblastic factors support tumor growth in bone metastases (Hensel and Thalmann 2016). Both bone and immune system are affected in cancer at several stages (Di Rosa 2009; Criscitiello et al. 2015; Walsh and Choi 2014). Already at a non‐cancer state, patients that will develop bone metastases have a high number of T cells in their bone marrow (Zhao et al. 2012; Di Rosa 2009). This could be due to the modulation of a pre‐metastatic niche for the arrival of tumor cells. Disseminated tumor cells (DTCs) residing in bone marrow do not respond to therapies when they are in a dormant state, setting the stage for a relapse later. But how is this dormancy maintained and what is the role of immune cells in the process? The induction and maintenance of dormancy are partly mediated through CD8+ T cells in the bone marrow (Stefanovic et al. 2013). The occurrence of bone metastasis happens through a loss of T‐cell function and is managed through complicated interactions between tumor and immune cells in the bone marrow. Transfer of tumor‐reactive bone marrow‐derived cells increases the number of CD8+ cells in peripheral blood in about half of breast cancer patients, and this increase is associated with a prolonged survival. The immune microenvironment in bone metastases can induce anti‐ and pro‐tumor effects. These effects are described in more detail in a recent review chapter (Kähönen et al. 2021). In this review, we also proposed a novel concept of osteoimmuno‐oncology (OIO), referring to interactions of tumor, immune, and bone cells in bone metastasis. Antitumor effects are mainly caused by T and B cells that are supported by the stromal production of cytokines in bone marrow (Walker et al. 2016). For example, helper T cells and cytotoxic T cells become activated by tumor‐secreted factors that polarize M1 Mφ macrophages to kill tumor cells. Pro‐tumor effects are caused by immunosuppressive cells, and bone marrow is generally considered to be an immunosuppressive microenvironment (Criscitiello et al. 2015). These immunosuppressive cells include for example Tregs and MDSCs (Walker et al. 2016). Tumor microenvironment produces many inflammatory factors that result in the expansion of these cells (Walker et al. 2016). Upregulation of the immunosuppressive phenotype can also happen by local cells such as mesenchymal stromal cells in bone microenvironment, which through TGFβ can increase the number of Tregs. Furthermore, the infiltration of Tregs into the primary tumor has been recently proposed to promote the metastatic potential of cancer cells (Koh and Kang 2012). Also, Mφ macrophages are educated at the metastatic site to M2 Mφ macrophages, for example by secretion of monocyte chemoattractant protein 1 (MCP‐1), which increases local immunosuppression (Walker et al. 2016). Importantly, M2 Mφ macrophages can facilitate tumor cell migration and invasion and protect cancer cells from antitumor effects of T cells. The macrophages create a tumor growth‐promoting microenvironment that supports the formation of metastases and partly induces resistance to chemotherapies. As the tumors grow in bone, the normal function of the RANK/RANKL/OPG‐pathway is disrupted, leading to an imbalance in bone formation and resorption. This may happen through T cells, which contribute to bone loss in MM and in bone metastasis (Di Rosa 2009). For example in MM, T cells stimulate RANKL and induce osteoclast‐mediated bone resorption. As described above, many immune cells have multiple roles in the regulation of early steps in bone metastasis, from the formation of metastases to the regulation of growth and inducing resistance to current therapies. This highlights the need for understanding these interactions better in order to develop new treatment options to prevent the formation and growth of bone metastases. In order to do so, valid preclinical models that can be used in such studies are needed. Because endocrine‐related cancers such as breast and prostate cancer metastasize to bone in high frequency, understanding the diverse effects of sex steroids on metastasis is essential. Sex steroids are steroid hormones that interact with the estrogen receptor (ER) and androgen receptor (AR). In bone metastases, all tumor, bone, and immune cells are affected by sex steroids. Breast cancer subtypes are immunogenically different. Studies of tumor‐infiltrating lymphocytes (TILs) and cancer genome sequences have shown that ER‐, HER2+, and triple‐negative (ER, progesterone receptor: PR, and HER2 negative) breast cancer (TNBC) are more immunogenic than ER+ and HER2‐ subtypes (Sugie 2018). However, immunogenicity has mainly been investigated from primary tumors excluding biopsies of bone metastases. At the moment, it is not well characterized if bone metastases differ in their immunogenic profile according to concurrent hormone receptor status. In hormone‐receptor positive patients, endocrine therapies are used as a first‐line treatment. Though effective against a primary tumor, these therapies can have harmful effects on the skeleton by increasing bone loss and therefore increasing the risk of fractures (Bedatsova and Drake 2019). Indeed, estrogens are major hormonal regulators of bone homeostasis, and studies have supported the hypothesis that estrogen deficiency in women leads to an activated T‐lymphocyte phenotype, which may further contribute to the observed bone loss (Adeel et al. 2013). Furthermore, studies on immunotherapy combinations with anti‐hormone compounds are under investigation to increase the efficacy of immunotherapies in breast cancer patients. If immunotherapies will be used to treat patients with bone metastases, their effects in the bone microenvironment may be different in patients treated with anti‐hormonal therapies compared to hormone‐naïve patients. Prostate cancer often progresses to a metastatic castration‐resistant stage (mCRPC) where the majority of patients develop metastases to the skeleton. For example bone targeting radium‐223 dichloride and DC‐based immunotherapy sipuleucel‐T have been approved for mCRPC (Logothetis et al. 2018). However, immunotherapies have not yet shown clear efficacy in prostate cancer, even though there are some promising reports (Antonarakis et al. 2020), and metastatic prostate cancer still remains incurable (Dong et al. 2019). The prostate tumor microenvironment is immunosuppressive and less likely to respond to immunotherapies. However, preclinical studies have suggested that local sex steroids may influence immune cells in the prostatic microenvironment as both ER and AR are expressed in prostate (Bousset et al. 2018). Androgens have been shown to have immunosuppressive effects while estrogens induce prostate inflammation with infiltration of various immune cells, including T cells, macrophages, and neutrophils (Yatkin et al. 2009; Bernoulli et al. 2008). To enhance novel therapeutic development against bone metastatic prostate cancer, a better understanding of interactions between sex steroids, bone microenvironment, and immune cells is needed. Different models to study bone metastasis have been recently reviewed (Simmons et al. 2015; Jinnah et al. 2018; Kähkönen et al. 2019). These models are of high need in order to develop new therapies to diminish formation of or treat already formed metastases in the skeleton (Simmons et al. 2015; Garcia et al. 2008). When developing such models, attention should be paid to clinical relevance and ideally, the models should feature important aspects of the human disease (Simmons et al. 2015). The bone microenvironment is unique and important for supporting tumor growth locally (Xia et al. 2012). Bone microenvironment contains many cell types including immune cells, fibroblasts, endothelial cells, blood and lymphoid cells, and importantly, bone cells and bone extracellular matrix (Xia et al. 2012). Bone metastasis models can be divided into three categories: spontaneous, systemic and local models (Garcia et al. 2008). Of these, spontaneous models where the tumors form in the primary organ and metastasize to bone, are very rare (Simmons et al. 2015; Xia et al. 2012; Kuperwasser et al. 2005). Genetically engineered mouse models (GEMMs) develop cancer in the primary organ and, importantly when considering IO drug development, the studies can be conducted in immunocompetent mice (Simmons et al. 2015). Many of these models have phenotypic and morphologic similarities to human disease. The disadvantage of this approach is the long development and validation of the models and that studies performed in the established models are very time‐consuming. The greatest obstacle in using GEMMs is typically a low rate of bone metastases in the mice (Xia et al. 2012). Because of this, most models utilize injection of cultured tumor cells (Simmons et al. 2015). The disadvantage of this approach is that it lacks the process of carcinogenesis and the tumors are typically formed from one‐cell clone, which does not fully recapitulate the heterogeneity in metastases observed in patients (Simmons et al. 2015). When tumor cells are injected orthotopically (i.e. breast cancer cells into the mammary fat pad or intraductally, prostate cancer cells into prostate) they can ideally recapitulate the process of metastatic spread from a primary site to distant metastatic sites, including bones (Simmons et al. 2015; Nogawa et al. 2005; McGovern et al. 2018). Whether the whole process occurs in mice depends mainly on the properties of the cell line used, and most cell lines do not form bone metastases when inoculated this way (Simmons et al. 2015). Because of this, many researchers utilize systemic models where cancer cells are inoculated into the left cardiac ventricle of the heart or intravenously into the tail vein (Simmons et al. 2015; Garcia et al. 2008; Xia et al. 2012; Buie et al. 2013). Generally, the intracardiac inoculation method is recommended in the case of lung‐seeking cells to increase metastases to skeleton (Simmons et al. 2015). Also, other systemic inoculation techniques such as inoculation through caudal arteries can be used (Kuchimaru et al. 2018). In all systemic models, metastases are typically formed to multiple organs, not only to bones. The ratio of bone metastases to metastases to other sites largely depends on the cell line used (Simmons et al. 2015). The last category of models is the so‐called bone growth models, where cancer cells are directly inoculated into the bone marrow (i.e. tibia or femur) (Simmons et al. 2015; Garcia et al. 2008; Buie et al. 2013). These models can be used to study cancer‐induced effects on bone, and for testing efficacy of compounds on tumors growing in bone microenvironment (Simmons et al. 2015; Garcia et al. 2008). Systemic and local bone metastasis models are clinically relevant because by the time of initial cancer diagnosis, many patients already have DTCs in their bone marrow, eventually forming clinically detectable metastases, and the models mimic clinical features observed in patients (Braun et al. 2005; Morgan et al. 2009). During recent years, many novel bone metastasis models have been established to provide tools for understanding better the biology behind the complex process of bone metastasis. Some of these models are highlighted in this paragraph. Many bone metastasis studies have been hindered by the low take rate of bone metastases with the cancer cell line of interest. To improve this, cells can be passaged in mice, i.e. inoculated to mice, then collected from the metastatic site, and re‐inoculated to new mice. With this approach, the tumor cells are “educated” in their metastatic microenvironment and/or a subset of cells capable of forming bone metastases is enriched, which usually increases the seeding and/or growth of these cells in the bone metastatic location. This has been done for several cell lines such as the MDA‐MB‐231 human breast cancer cell line, resulting in bone‐seeking cell lines B02 (Garcia et al. 2008) and (SA) (Moseley et al. 2018) that after passaging metastasize to bone after systemic injection (Garcia et al. 2008), and for the lung cancer cells line 786‐O that after intracardiac inoculation results in 786‐O(HM) metastatic line, producing osteolytic bone metastases with a higher incidence compared to the parental cell line (Strube et al. 2010). Patient‐derived xenograft (PDX) models have been widely used with subcutaneous implantation. Recently, PDX models have also been applied to bone metastatic setting. One of the first examples is the PCSD1 (Prostate Cancer San Diego 1), a patient‐derived intra‐femoral xenograft model that features mixed osteolytic and osteoblastic lesions (Raheem et al. 2011). After this model, others have continued to establish similar models. Different kinds of bone scaffold models utilizing the so‐called “humanized bone” have also been established. These models can be used to study interactions between human bone and human tumor cells (McGovern et al. 2018; Nemeth et al. 1999). In these studies, for example fragments of fetal human bone or bone obtained from the femoral head of adult patients (Amanatullah et al. 2017) can be implanted to mice subcutaneously, and the tumor cells can then migrate to grow in the bone fragments (Simmons et al. 2015; Nemeth et al. 1999
6
Preclinical Osteoimmuno‐Oncology Models to Study Effects of Immunotherapies on Bone Metastasis
6.1 Introduction to Metastasis and Use of Preclinical Models
6.2 Bone Metastatic Microenvironment
6.2.1 Introduction to Bone Biology
6.2.2 Immune Cells in the Bone Marrow
6.2.3 Osteoimmunology
6.2.4 Immunotherapies and Bone Safety
6.2.5 Vicious Cycle of Bone Metastasis
6.2.6 Immune Cells in Regulating Metastasis to Bone
6.2.7 Effects of Estrogens and Androgens on Cancer, Bone, and Immune System
6.3 Bone Metastasis Models
6.3.1 Conventional Models to Study Bone Metastasis
6.3.2 Novel Bone Metastasis Models
Stay updated, free articles. Join our Telegram channel
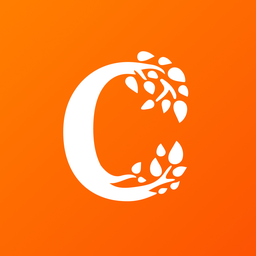
Full access? Get Clinical Tree
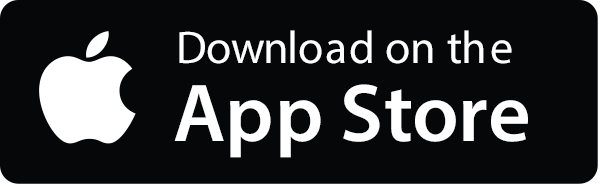
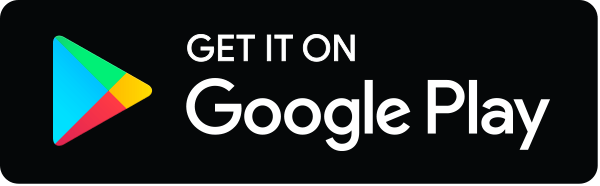