Zhuo Li, Xiao Yang, and Xiaomin Song Department of Pharmacology, BeiGene (Beijing) Co., Ltd., Beijing, China While targeting tumor‐intrinsic oncogenic factors (i.e. aberrant driver events in tumor cells) remains a key approach to targeted therapies, a new era of cancer immunotherapy (also known as immuno‐oncology, I‐O) has arrived. Immunotherapies have demonstrated unprecedented rates of long‐term antitumor responses in patients with a variety of cancers (Ribas and Wolchok 2018). Clinically approved I‐O agents targeting inhibitory immune receptors (also known as immune checkpoints) CTLA‐4 and PD‐1/PD‐L1 emphasize on tumor–stromal interactions, especially the tumor‐immune cell crosstalk (Pardoll 2012; Kruger et al. 2019). Immune competent mouse with transgenic or knock‐in of human genes provides a valuable genetically engineered mouse model (GEMM) to study checkpoint‐mediated immunity in an intact murine immune system (Kersten et al. 2017). However, inherent differences between human and mouse immune systems and the absence of mouse homologues of some human targets may limit studies on immune‐dependent antitumor activities in GEMM models (Mestas and Hughes 2004; Zitvogel et al. 2016). Therefore, tremendous effort has been devoted to “humanize” the murine immune system, through reconstitution of human immune cells in immunocompromised mice (De La Rochere et al. 2018; Curran et al. 2020). The biggest limitation of human immune cell engraftment has been the lack of mouse strains that could support and maintain human immune cell growth. The nude mouse was first discovered and characterized in the 1960s and found absent of thymus and T lymphocytes (Flanagan 1966; Pantelouris 1968). The nude mice, as the first immunocompromised strain defective in adaptive immune responses, have been widely used in human tumor xenograft transplantation. However, the intact innate immunity in these nude mice rejects engraftment of nontumor tissues such as human bone marrow (BM) suspensions (Ganick et al. 1980). The first breakthrough came with the discovery of immunocompromised mice harboring the Prkdcscid mutation. In 1983, Bosma et al. descried a strain that carries a spontaneous, autosomal‐recessive mutation in the protein kinase, DNA‐activated, catalytic polypeptide (Prkdc) gene. This strain lacks both functional T and B lymphocytes, mimicking the clinical phenotypes of human severe combined immunodeficiency (SCID) and therefore subsequently named as SCID mice (Bosma et al. 1983). PRKDC is essential for resolving DNA double‐strand breaks during variable, diversity, and joining [V(D)J] recombination during the development of T and B cells (Lieber et al. 1988; Blunt et al. 1996; Taccioli et al. 1998). Successful engraftment of human peripheral blood mononuclear cells (PBMCs) (Mosier et al. 1988), BM‐derived HSPCs (Lapidot et al. 1992), and fetal tissues (McCune et al. 1988) have been reported. However, SCID mice still maintain a functional innate immune system; NK cells are present and could result in rejection of implanted cells. At around the same time, the nonobese diabetic (NOD) mice was discovered by Makino et al. (Makino et al. 1980). The NOD strain was characterized to have impaired innate immunity associated with NK, macrophage, and dendritic cell malfunctions (Kataoka et al. 1983; Kikutani and Makino 1992; Anderson and Bluestone 2005). Eventually, the SCID mutation was backcrossed onto the NOD strain background. This resulted in the creation of the NOD SCID mice by Shultz et al. (Shultz et al. 1995). The NOD SCID strain demonstrated deficiencies in both the innate and adaptive immunity (Shultz et al. 1995), and has served since then as a preferred model by many investigators for human immune cell engraftment. Nevertheless, the NOD SCID strain still has limitations. The mice were prone to developing thymomas and demonstrated “leakiness” – the eventual production of B and/or T cells over time due to incomplete V(D)J recombination defects (Shultz et al. 1995; Prochazka et al. 1992; Bosma 1992). There are residual NK activities in this NOD SCID strain, thus compromising engraftment efficiency (Yoshino et al. 2000). Interleukin‐2 receptor γ chain (IL‐2Rγ) gene encodes a cytokine receptor subunit constituting the receptor complexes for IL‐2, IL‐4, IL‐7, IL‐9, IL‐15, and IL‐21, and is critical for innate and adaptive lymphoid development (Sugamura et al. 1996). IL‐2Rγ‐deficient mice demonstrate markedly reduced NK‐, T‐, and B‐cell numbers and functions (Cao et al. 1995; DiSanto et al. 1995). Crossing IL‐2Rγnull mice with the NOD SCID mice results in the NOD SCID IL‐2Rγnull strain that are severely immunodeficient due to the lack of mature T, B, and NK cells, impaired cellular functions from the myeloid lineage, and prevention of cytokine signaling through multiple receptors (Ito et al. 2002; Shultz et al. 2005). These NOD SCID IL‐2Rγnull mice (e.g. NSG with a complete null mutation of IL‐2Rγ, NOG with a truncated intracellular signaling domain of IL‐2Rγ (Nagatani et al. 2019)) demonstrate improved engraftments of both tumoral and nontumoral human tissues. The recombination activating gene 1 (Rag1) and gene 2 (Rag2) play important functions in the initiation of V(D)J rearrangement (Schatz and Ji 2011). Knock‐out of Rag1 or Rag 2 resulted in defective primary lymphoid organs that do not contain mature T and B lymphocytes (Mombaerts et al. 1992; Shinkai et al. 1992). By crossing IL‐2Rγnull mice with strains carrying the Rag1 or Rag2 deficiency, the NRG and BRG mice have been established (Pearson et al. 2008a; Baenziger et al. 2006). The NRG and BRG mice have also been used for human immune cell engraftment and are more resistant to irradiation and genotoxic drugs than mice with a SCID mutation in the DNA repair enzyme Prkdc. Commonly used immunocompromised mouse strains for I‐O research are summarized in Table 7.1. As the demand for humanized mouse models increase, newer immune‐deficient strains have been established for specific experimental needs. Strain variants engineered based on the Prkdcscid IL‐2Rγnull or Rag1/2null IL‐2Rγnull mutations are summarized in Table 7.2. Xenogeneic Graft‐Versus‐Host Disease (xGvHD) usually develops a few weeks after human PBMC engraftment. This phenotype is attributed to the mismatch of major histocompatibility complex (MHC) between human T cells and mouse cells (Sanmamed et al. 2016). As a result, engrafted mature human T cells recognize murine xeno‐antigens presented by murine MHC, target mouse organs and tissues, and ultimately kill the animals (King et al. 2009). To address this issue, mouse MHC class I and/or II knockout strains have been established. The resulting strains demonstrated an attenuated xGVHD development post human PBMC transplantation (Covassin et al. 2013; Brehm et al. 2019). Table 7.1 Commonly used immunocompromised mouse strains. Table 7.2 Emerging strain variants for HIS reconstitution. In addition, mice engineered to express human HLA genes have also been established. An absence of human leukocyte antigen (HLA) molecules resulted in human pre‐T cells being educated and selected on mouse thymus and MHC. Human HLA class I or class II transgenic mice demonstrated high engraftment of HLA class‐restricted immune cells after CD34+ humanization, supporting studies on human T‐cell repertoire and responses (Shultz et al. 2010; Covassin et al. 2011). Besides T cells, multiple immune cell populations, such as NK cells and monocytes, also play crucial functions during tumor progression and are now being actively explored for immunotherapies. The poor survival of NK cells and the myeloid compartment has long been a limitation of humanized mouse models due to the lack of key human hematopoietic growth factors and cytokines. To solve this problem, newer immunocompromised strains harboring knockin of human cytokine genes have been developed. For example, the NSG‐IL15 and hIL‐15 NOG strains with transgenic human IL‐15 expression, demonstrate enhanced NK‐cell development and survival in mouse blood, spleen, and BM (Brehm et al. 2018; Katano et al. 2017). Mice with knock‐in of human IL‐15 and SIRPα (SRG‐15) have also been generated based on the BALB/c Rag2−/− IL2rgc−/− strain (Herndler‐Brandstetter et al. 2017). Signal‐regulatory protein alpha (SIRPα) binds to human CD47 and produces anti‐phagocytic signals which inhibit murine macrophages from engulfing engrafted human cells (Takenaka et al. 2007). These SRG‐15 mice demonstrated improved development and functional maturation of circulating and tissue resident human NK and CD8+ T cells and promoted the development of tissue‐resident innate lymphoid cell (ILC) subsets (Herndler‐Brandstetter et al. 2017). The NOG‐EXL strain is engineered to express both human granulocyte/macrophage colony‐stimulating factor (GM‐CSF) and IL‐3, and it supports increased reconstitution and differentiation of the human myeloid lineage compared to its parental NOG strain (Fukuchi et al. 1998; Ito et al. 2013). Similarly, the triple transgenic NSG‐SGM3 mice expressing human GM‐CSF, IL‐3 and Kit ligand (KITLG; also known as stem cell factor, SCF) support improved reconstitution of myeloid lineages and regulatory T‐cell populations compared to its parental NSG strain (Billerbeck et al. 2011). These NSG‐SGM3 mice were demonstrated with improved engraftment of diverse hematopoietic lineages and primary AML samples (Billerbeck et al. 2011; Wunderlich et al. 2010). Another model, the MISTRG mice, which express human GM‐CSF, IL‐3, SIRPα, macrophage colony‐stimulating factor (M‐CSF) and thrombopoietin (TPO), was designed to support a greater level of human immune cell reconstitution, particularly in the myeloid compartment (Rongvaux et al. 2014). However, the MISTRG strain develops severe anemia due to poor erythropoiesis and results in short lifespan and was eventually discontinued commercially (Rongvaux et al. 2014). Recently, a c‐kit mutation, which suppresses mouse stem cell self‐renewal and differentiation, has been introduced into NSG mice and the resulting strains, NBSGW and NSGW41, demonstrate multilineage human hematopoietic reconstitution without preconditioning irradiation (Cosgun et al. 2014; McIntosh et al. 2015). Hanazawa’s group generated human IL‐6‐expressing NOG mice (NOG‐hIL‐6 transgenic mice) allowing the spontaneous development and maintenance of monocytes/macrophages by the engraftment of HSPCs. The immunosuppressive myeloid cells detected in NOG‐hIL‐6 transgenic mice bearing tumors were CD163+ TAM like cells, not HLA‐DRlo/− MDSC like cells, thus only partly enabled reconstitution of the tumor microenvironment () (Hanazawa et al. 2018). Two types of human immune cells are used to engraft immunocompromised mice with functional human immune system (HIS), (i) PBMCs, (ii) CD34+ hematopoietic stem and progenitor cells (HSPCs). These cells are mainly used in three models, namely (i) Hu‐PBMC model, (ii) Hu‐CD34+ HSPC model, and (iii) bone marrow–liver–thymus (BLT) model. The BLT model involves surgical implantation of fragments of human fetal liver and thymus under the kidney capsule of sub‐lethally irradiated immunocompromised mice, followed by an intravenous injection of autologous HSPCs. The BLT model is covered in Chapter XYZ and thus will not be discussed here. The other two models, Hu‐PBMC and Hu‐CD34+ HSPC, will be described in detail (Figure 7.1, Table 7.3). The Hu‐PBMC model, also known as human peripheral blood leukocyte (Hu‐PBL) model, provides a simple and cost‐effective approach of humanization. Reconstitution of immunocompromised mice with human PBMCs allows the study of HIS, particularly the CD8+ T‐cell population, and its responses to cancer in an in vivo model. PBMCs originate from hematopoietic stem cells (HSCs) and are constituted by cells from both the lymphoid and myeloid lineages (Autissier et al. 2010; Kleiveland 2015). About 70–90% of PBMCs are lymphocytes, with the most significant portion being CD3+ T cells (45–70%), followed by CD19+ B cells (5–15%), and NK cells (5–10%). T and B cells in PBMCs isolated from healthy donors largely exist in a naïve state and once activated, they will induce cell‐mediated and humoral immune responses, respectively. Monocytes account for 10–30% of cells in PBMC. They circulate in the blood and continually migrate into peripheral tissues. Upon stimulation, monocytes differentiate into dendritic cells and macrophages, which could mediate both the innate and adaptive immune responses. Dendritic cells (1–2%) and HSCs (0.1–0.2%) are also found at low frequencies in PBMCs. Figure 7.1 Generation of different HIS mouse models. Hu‐PBMC model: human peripheral blood mononuclear cells (PBMCs) are isolated from peripheral whole blood and engrafted in immunocompromised mice via intravenous (IV), intraperitoneal (IP), or subcutaneous (SC) route. Hu‐CD34+ HSPC model: human CD34+ HSPCs are obtained from granulocyte colony stimulating factor (G‐CSF) mobilized peripheral blood, bone marrow, umbilical cord blood or fetal liver, and subsequently injected into adult or newborn immunocompromised mice via IV, IP, intrafemoral (IF), intracardiac (IC), or intrahepatic (IH) route. Hu‐BLT model: human fetal liver and thymus fragments are implanted under the renal capsule of adult immunocompromised mice, and autologous CD34+ HSPCs derived from the same fetal liver tissue are injected by IV. Obtaining PBMC samples is relatively straightforward. With appropriate Institutional Review Board (IRB) approval, peripheral whole blood can be collected by venipuncture from volunteers following informed consent and local regulatory requirements. From healthy blood, PBMC yield ranges between 0.5 and 2 × 106 cells per mL blood (Bittersohl and Steimer 2016). The most common method to isolate PBMCs from whole peripheral blood is density gradient centrifugation (Ulmer et al. 1984). This process separates different cell populations according to their varying densities throughout a density gradient medium. After centrifugation, the PBMC fraction appears as a thin white layer at the interface between the plasma and the medium. Maintaining proper temperatures (experimental procedures, reagent and sample storage, etc.) and reducing processing time could help improve PBMC viability and recovery and ensure reliability and consistency of downstream applications. Table 7.3 Commonly used human immune system (HIS) models. Isolated PBMCs can be engrafted via intravenous (IV), intraperitoneal (IP), or subcutaneous (SC) co‐injection with mixed human tumor cells. The intrasplenic route has also been documented (Steyaert et al. 2007) but is more time consuming and requires surgical proficiency. Detailed methods for human PBMC and HSPC engraftment have been previously reviewed (Pearson et al. 2008b). Despite heterogenous immune cell populations found in PBMCs prior to engraftment, T cells are the primary subpopulation that resides and remains functional in the recipient mouse. Human T cells can be maintained for up to four to six weeks, with an activated memory and effector phenotypes (Pearson et al. 2008b; King et al. 2008). B cells and most innate immune cells, however, tend to die off within the first few days of engraftment, likely due to dominant expansion of T cells and lack of appropriate human cytokines required for survival (Curran et al. 2020). Commercially available immunocompromised strains such as NOD SCID and NOD/SCID/IL‐2Rγ knockout (e.g. NSG, NOG) have been proved to be efficient at engrafting mature human T cells from PBMCs. The availability of healthy PBMC donors and relatively simple and cost‐effective cell isolation procedures makes the Hu‐PBMC model a powerful tool for I‐O studies. Robust and reproducible T‐cell responses, combined with wide distribution of T cells in multiple organs, allow effective preclinical evaluation of immunomodulatory therapeutics in vivo. A single donor alone would provide enough PBMCs for an in vivo study with dozens of mice, minimizing inter‐ and intra‐group variations resulted from different donors (Li et al. 2019). Moreover, rapid humanization process prepares readily usable humanized mice within days of engraftment. The major drawback of the Hu‐PBMC model is development of xGvHD, usually at a few weeks post human PBMC engraftment. The induction of xGvHD is due to MHC mismatch between human T cells and mouse cells (Sanmamed et al. 2016). The onset and severity of xGvHD correlate with the expansion of activated human T cells and attacking mouse tissues in the murine host (Ehx et al. 2018). As a result, the experimental window of the Hu‐PBMC model is usually limited to four to six weeks (De La Rochere et al. 2018). To solve this issue, researchers have established newer strains of mice deficient in MHC class I and/or II and demonstrated delayed xGvHD onset and increased survival (Covassin et al. 2013; Brehm et al. 2019). Preconditioning irradiation of NSG mice before injecting PBMCs is not required as mature human leukocytes are injected. However, it has been reported that a sublethal dose of irradiation facilitates engraftment and colonization of human PBMCs in the murine host; this leads to faster and enhanced T‐cell expansion but also accelerates xGvHD, resulting in a shorter survival time compared to nonirradiated mice receiving PBMCs (King et al. 2009; King et al. 2008). Considering the genetic diversity among human population (e.g. HLA type and expression), donor‐to‐donor difference in engraftment kinetics, and variations in progression and severity of xenogeneic T‐cell activation, investigators are encouraged to prescreen a panel of PBMC donors and set up additional studies to understand how therapeutics work across multiple genetically diverse donors (Li et al. 2019; Verma et al. 2019). Human HSPCs have the potential to differentiate into all human hematopoietic lineages, thereby serving as a reliable source for HIS reconstitution in immunocompromised mice. CD34 is a transmembrane phosphoglycoprotein protein and a cell–cell adhesion factor (Krause et al. 1996; Lanza et al. 2001). It was first described on HSCs by Civin et al. (1984) and Tindle et al. (1985) independently, and is considered as a key surface marker for HSPCs. Although combined markers have been proposed to precisely define HSPCs, the single, less discriminating marker CD34 could still capture most cells with the long‐term repopulating activities (Hogan et al. 1997; Kawashima et al. 1996; Gao et al. 2001
7
Humanized Mouse Models for Cancer Immunotherapy: A Focus on Human PBMC and HSPC Reconstitution
7.1 Immunocompromised Mice
7.1.1 An Evolving History of Mouse Strains
7.1.2 Emerging Strain Variants
Strain name
Altered genes
Genetic background
Year established
Immunological characteristics
Additional notes
Reference
Commercial vendor
Athymic nude, nu/nu
Foxn1
Homozygous Foxn1 mutant spontaneously arose in an albino strain. Foxn1 homozygous null also available in C57BL/6 and BALB/c background
1966
Flanagan (1966) and Pantelouris (1968)
Multiple suppliers
SCID (C.B17‐scid)
Prkdc
C.B‐Igh‐1b/IcrTac‐Prkdcscid
1983
Increased sensitivity to DNA damaging agents, such as chemotherapy and irradiation, due to Prkdc mutation. Lower irradiation tolerance compared to mice with a knockout of Rag1 or Rag2
Bosma et al. (1983)
Multiple suppliers
NOD SCID, NOD scid
Prkdc
NOD.CB17‐Prkdcscid/J
1995
Shultz et al. (1995)
Multiple suppliers
NSG (NOD.Scid.Gamma)
Prkdc, Il2rg
NOD.Cg‐Prkdcscid Il2rgtm1Wjl/SzJ
2005
Shultz et al. (2005)
The Jackson Laboratory
NOG (NOD.Gamma)
Prkdc, Il2rg
NOD.Cg‐Prkdcscid Il2rgtm1Sug/JicTac
2002
Ito et al. (2002)
Taconic Biosciences
NRG (NOD.Rag.Gamma)
Rag1, Il2rg
NOD.Cg‐Rag1tm1Mom, Il2rgtm1Wjl/SzJ
2008
Pearson et al. (2008a)
The Jackson Laboratory
BRG (BALB/c.Rag.Gamma)
Rag2, Il2rg
C.Cg‐Rag2tm1Fwa Il2rgtm1Sug/JicTac
2004
Baenziger et al. (2006) and Traggiai et al. (2004)
Taconic Biosciences
Strain name
Genetic alterations
Human immune cell engraftment model
Remarks
Reference
Commercial vendor
NOD SCID
Prkdc null
Hu‐PBMCs, Hu‐HSPCs, Hu‐BLT
—
Shultz et al. (1995)
Multiple suppliers
NSG (NOD.Scid.Gamma)
Prkdc null, IL2rg null
Hu‐PBMCs, Hu‐HSPCs, Hu‐BLT
Shultz et al. (2005)
The Jackson Laboratory
NOG (NOD.Gamma)
Prkdc null, IL2rg null
Hu‐PBMCs, Hu‐HSPCs, Hu‐BLT
Ito et al. (2002)
Taconic Biosciences
NRG (NOD.Rag.Gamma)
Rag1 null, IL2rg null
Hu‐PBMCs, Hu‐HSPCs, Hu‐BLT
Pearson et al. (2008a)
The Jackson Laboratory
BRG (BALB/c.Rag.Gamma)
Rag2 null, IL2rg null
Hu‐PBMCs, Hu‐HSPCs
Baenziger et al. (2006) and Traggiai et al. (2004)
Taconic Biosciences
MHC Class I‐null, MHC I/II KO
Mouse MHC Class I, or Class 1/2 knockout based on NSG strain
Hu‐PBMCs, Hu‐HSPCs
Delayed xGVHD post human PBMC transplantation
High hCD45+ cell engraftment (Stock No: 023848)
Useful for studying mechanisms for xGVHD
Covassin et al. (2013)and Brehm et al. (2019)
The Jackson Laboratory
HLA Class I‐A2 Transgenics
Human HLA Class I‐A2 knock‐in based on NSG strain
Hu‐PBMCs, Hu‐HSPCs
Shultz et al. (2010)
The Jackson Laboratory
HLA Class II Transgenics
Human HLA Class II knock‐in based on NSG strain
Hu‐PBMCs, Hu‐HSPCs
Covassin et al. (2011)
The Jackson Laboratory
NSG‐IL15, hIL‐15 NOG
Human IL‐15 knock‐in based on NSG or NOG strain
Hu‐PBMCs, Hu‐HSPCs
Brehm et al. (2018) and Katano et al. (2017)
The Jackson Laboratory, Taconic Biosciences
SRG‐15
Human IL‐15 and SIRPα knock‐in on BRG strain
Hu‐HSPCs
Herndler‐Brandstetter et al. (2017)
—
NOG‐EXL
Human GM‐CSF and IL‐3 knock‐in based on NOG strain
Hu‐PBMCs, Hu‐HSPCs, Hu‐BLT
Fukuchi et al. (1998) and Ito et al. (2013)
Taconic Biosciences
NSG‐SGM3
Human GM‐CSF, IL‐3 and KITLG/SCF knock‐in based on NSG strain
Hu‐PBMCs, Hu‐HSPCs, Hu‐BLT
Billerbeck et al. (2011) and Wunderlich et al. (2010)
The Jackson Laboratory
MISTRG
Human GM‐CSF, IL‐3, M‐CSF, TPO and SIRPα expression based on BRG strain
Hu‐HSPCs
Rongvaux et al. (2014)
Discontinued commercially
NBSGW, NSGW41
c‐kit mutation based on NSG strain
Hu‐HSPCs
Cosgun et al. (2014) and McIntosh et al. (2015)
The Jackson Laboratory
7.2 Human Immune System (HIS) Models
7.2.1 Hu‐PBMC Model
Hu‐PBMC
Hu‐CD34+ HSPC
Source of human immune cells
Human peripheral blood mononuclear cells (PBMCs)
Human CD34+ hematopoietic stem and progenitor cells (HSPCs) from umbilical cord blood (UCB), bone marrow (BM), fetal liver (FL), or G‐CSF‐mobilized peripheral blood
Commonly used mouse strain
NOD SCID, NSG/NOG, NRG/BRG
NSG/NOG, NRG/BRG, other variants based on these mice
Route of injection
i.v., i.p., or s.c. admixed with tumor cells
i.v., i.p., or intrafemoral (adult mice); i.v., i.p., intracardiac, or intrahepatic (newborn mice)
Sublethal γ‐irradiation preconditioning
Usually not required for PBMC engraftment in NSG/NOG; may be needed for better human tissue engraftment in other strains
Yes (except for the c‐kit null variants)
Human immune cells engrafted
High level of functional T‐cell populations, retaining immune memory and antigen specificity from donors
Differentiation of multiple hematopoietic lineages at varying degrees
Expected engraftment efficiency
20‐30% hCD45+ cells in mouse peripheral blood
>25% hCD45+ cells in mouse peripheral blood
Time for immune cell reconstitution
Rapid (three to five days)
Long (three to four weeks)
Experimental length
Short (four to eight weeks), limited by xGVHD
Long (up to seven to eight months), relatively stable immune cell reconstitution, no obvious xGVHD
Cost
Low
High
Technical complexity
Low
Moderate to high
Accessibility to donor
Easy
Hard
Applications in I/O research
Human tumor immunology, preclinical efficacy and safety assessment of agents targeting T‐cell functions (e.g. immune checkpoint inhibitors, bispecific T‐cell engager, CAR‐T therapy)
Additional remarks
Knockout mice for MHC class I and/or MHC class II have been established in order to delay onset of xGVHD
7.2.2 Hu‐CD34+ HSPC Model
Stay updated, free articles. Join our Telegram channel
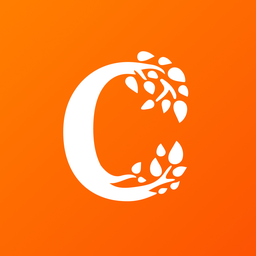
Full access? Get Clinical Tree
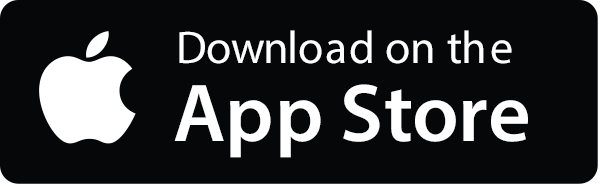
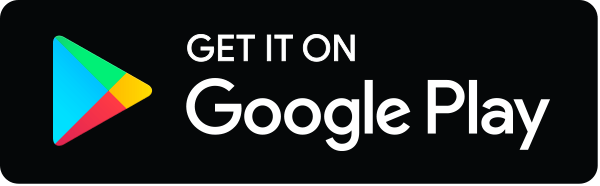