Vaidehi V. Paranjape1 and Fernando Garcia‐Pereira2 1 Department of Small Animal Clinical Sciences, Virginia‐Maryland College of Veterinary Medicine, Virginia Tech University, Blacksburg, Virginia, USA 2 Pet Urgent Response and Emergency, Jacksonville, Florida, USA The liver is centrally located between the diaphragm and abdominal viscera and receives 20–30% of total cardiac output (CO) via the portal vein and hepatic artery. The liver plays a vital role in homeostasis and is responsible for the biotransformation and excretion of endogenous and exogenous substances. Significant exogenous physiologic support may be necessary for patients with clinically relevant hepatic dysfunction. In addition, consideration must be given to anesthetic and adjunct drug selection to avoid delayed recovery. Anesthetic and surgical procedures involving the liver may cause ischemia and reperfusion injury, inducing acute and chronic hepatic dysfunction. Hence, it is crucial to maintain adequate hepatic blood flow and oxygen delivery during general anesthesia. Clinical application of anatomic and physiologic knowledge is necessary to optimize anesthetic management during hepatic and extrahepatic biliary disease interventions. The liver is the largest gland in the body, comprising 1.5–4% of total body weight, and is anatomically and physiologically similar among species. The liver can be divided into four main lobes (left, right, quadrate, and caudate lobes). The right and left lobes may be further subdivided into separate lateral and medial lobes. A gallbladder is present in most domestic species with the exception of the horse and the rat, in which large bile ducts compensate for the absence of the gallbladder. In some species, the bile duct terminates directly on the duodenum (e.g., canine and bovine), but in others, it shares a common duct with the pancreas (e.g., cat, horse, and small ruminants). This can be of importance, as some hepatobiliary diseases can also affect the pancreas of those species [1]. The hexagonal hepatic lobule is the basic anatomic unit of the liver. Cylindrical plates of hepatocytes are separated by venous sinusoids (fenestrated capillaries) and radiate around a central vein like spokes in wheel. The portal triad (hepatic arterioles, portal venules, bile canaliculi, lymphatics, and nerves) is present at each corner of the hexagon. The perisinusoidal space (or space of Disse) lies between the sinusoids and hepatocytes, containing plasma and allowing hepatocytes to absorb nutrients and waste products. Macrophage‐derived Kupffer cells line the perisinusoidal space (Fig. 40.1). These cells have phagocytic function and are active in hepatic inflammatory responses. The hepatic arterial (HA) blood enters the sinusoid either directly or through the peribiliary capillary plexus and mixes with portal venous blood in the low‐pressure sinusoid microvasculature. Figure 40.1 Structures of the liver lobule and liver acinus. Source: Du et al. [2], with permission. Although the hepatic lobule is the traditional organizational unit of the liver, a more logical functional unit is the acinus, which comprises three different zones (Fig. 40.2). Zone 1, known as the periportal area is centered around the portal triad, making it oxygen rich due to its close proximity with the hepatic artery. Zone 1 is most adaptable to hemodynamic stressors, least susceptible to necrosis, and first to undergo regeneration. The hepatocytes in this zone contain many mitochondria, and most oxidative metabolic processes occur here. Zone 2 is a transitional zone between zones 1 and 3. Zone 3 is the pericentral zone, located in close proximity to the central vein (centrilobular), where blood leaves the organ to join the central circulation. This zone has the lowest oxygen tension, is most susceptible to hemodynamic and toxic stress, and is the last to regenerate. The hepatocytes in this zone contain a large amount of smooth endoplasmic reticulum and microsomal enzyme activity, and therefore play a major role in drug detoxification, or Phase I and II metabolism. Afferent blood supply to the liver is composed of both arterial and portal venous blood and all the blood exits the liver via the hepatic veins. The arrangement of hepatic structures is centered on the caudal vena cava and portal vein. The portal vein supplies 75% of the hepatic blood flow (HBF) and is formed by blood returning from the splanchnic circulation, including most of the gastrointestinal tract and spleen (Fig. 40.3). The splenic vein contribution to the portal blood is rich in pancreatic hormones and cytokines, while the superior mesenteric vein brings nutrients, toxins, and bacteria that are absorbed from the gastrointestinal tract. The HA provides the remaining 25% of HBF; however, it supplies 45–50% of the liver’s oxygen requirements. Although the portal vein carries blood with a lower oxygen content than the HA, its contribution to the organ’s oxygenation is significant due to the large volume of blood that it delivers [4]. HBF is regulated via intrinsic and extrinsic mechanisms. The HA supplies arterial blood via a high‐pressure system and mean pressure in the HA is similar to that in the aorta. The HA possesses α1‐, α2‐, and β2‐adrenergic receptors. Sympathetic activation constricts HA smooth muscle, increases arterial resistance, and reduces blood flow. β‐Adrenergic stimulation vasodilates hepatic arterioles, decreases vascular resistance, and increases flow through the HA. However, this effect may be blocked by non‐selective β‐adrenergic receptor antagonists administered to decrease portal pressure in human patients with liver cirrhosis [5]. In contrast, the portal vein contains α‐adrenergic but not β2‐adrenergic receptors [6]. Minor changes in hepatic venous tone and hepatic venous pressure can result in large changes in hepatic blood volume, favoring the liver’s ability to act as a blood reservoir. For example, during hemorrhage, hepatic venous pressure decreases, shifting blood into the central venous circulation and augmenting circulating blood volume. Blood loss can be reduced during liver surgery by lowering the central venous pressure, thereby reducing hepatic venous pressure and hepatic blood volume. The liver’s high blood flow is due to low vascular resistance in the portal vein. Normally, the portal vein pressure is about 7–10 mmHg. However, with hepatocellular injury, fibrous tissue can form in the affected areas, impeding blood flow and causing portal hypertension [6]. An increase in portal pressure can promote neovascularization of splanchnic vasculature and development of acquired portosystemic shunts. An increase in portal flow must be compensated such that portal venous pressure is minimally affected. In order to preserve adequate sinusoidal perfusion pressure while maintaining low portal venous pressure, HBF is regulated by mechanisms targeting HA inflow, portal venous inflow, and the interrelationship between HA and portal venous inflow circuits [7]. Several intrinsic mechanisms are responsible for maintaining adequate sinusoidal perfusion pressure while maintaining low portal venous pressure, including (1) low basal resistance, (2) distensible pre‐ and post‐sinusoid resistance sites, (3) the myogenic constrictive response of the HA, (4) the HA response to oxygen tension or pH of portal venous blood, (5) the highly compliant hepatic vasculature, and (6) the hepatic artery buffer response (HABR) [8]. These unique mechanisms represent the ability of the hepatic artery to produce compensatory flow changes in response to changes in portal venous flow. The HABR is mechanistically associated with adenosine because as portal blood flow decreases, adenosine accumulates, causing vasodilation of nearby hepatic arterioles. This dilation increases arterial blood flow and maintains hepatic perfusion [8]. Other vasoactive mediators such as nitric oxide, carbon monoxide, and hydrogen sulfide may be potential candidates to affect HA flow and contribute to the HABR. Moreover, sensory innervation (neurokinin‐1) and sensory neuropeptides (calcitonin gene‐related peptide) may also be related to HABR [7]. Figure 40.2 A. Microanatomy of the hepatic parenchyma within a hepatic lobule: Zone 1, periportal; zone 3, pericentral; zone 2, between zones 1 and 3. B. A schematic representation of a liver acinus and the corresponding zonation of several metabolic processes across the acinus. The gradients depicted below the scheme pertain to both essential hemostasis and metabolic processes along the acinus. Source: Trefts et al. [3], with permission. Figure 40.3 The splanchnic circulation. Source: Gelman and Mushlin [4], with permission. The liver performs numerous functions affecting all systems of the body (Table 40.1). It plays a major role in protein synthesis and degradation, including albumin, coagulation proteins, and numerous peptides. Glycogen storage, nutrient metabolism, detoxification, and excretion of endogenous waste products and xenobiotics are other important functions. Normally, plasma protein availability is abundant relative to daily consumption. Albumin is the most abundant protein produced by the liver and is involved in several homeostatic processes. Notably, albumin is a major contributor to plasma oncotic pressure. Albumin production is regulated (via feedback) in part through plasma oncotic pressure in addition to its plasma concentration [10]. Albumin is a low‐affinity/high‐capacity major plasma transport protein, and it plays a significant role in drug binding of acidic anesthetic drugs (e.g., barbiturates). In contrast, alpha‐1 acid glycoprotein (AAG) represents a relatively smaller percentage of total plasma proteins. It is considered a high‐affinity/low‐capacity plasma protein, and mainly binds to basic drugs (e.g., local anesthetics). In most disease states such as inflammation, infection, and neoplasia, AAG levels have been shown to increase in humans and animals. This promotes the utility of AAG as a clinical biomarker for disease diagnosis and prognosis [11]. Table 40.1 Pertinent functions of the liver. Source: Adapted from Mulaikal and Emond [9]. RAAS, renin–angiotensin–aldosterone system; HABR, hepatic artery buffer response. Several coagulation factors, such as fibrinogen (factor I), prothrombin (factor II), factors V, VII, IX, X, XI, XII, and XIII, prekallikrein, and high molecular weight kininogen are synthesized or activated by the liver. The liver is also responsible for the synthesis of proteins that modulate coagulation, such as plasminogen, plasminogen activator inhibitor‐1, α2‐antiplasmin, antithrombin, and proteins C and S. Furthermore, it performs vitamin K‐dependent carboxylation of prothrombin, factors VII, IX, and X, and proteins C and S [9]. The enzymatic systems involved in the biotransformation of substances are generally localized to the liver. However, other tissues, such as the lungs, kidneys, and gastrointestinal tract, have significant metabolic capabilities for some drugs. The route of administration and subsequent drug distribution to these sites must be considered when administering sedatives, analgesics, and anesthetics [12]. First‐pass hepatic metabolism significantly reduces the bioavailability of several drugs, especially after enteral, and in some species rectal, administration. Xenobiotic biotransformation can be divided into Phase I (oxidation, reduction, or hydrolysis) and Phase II (conjugation) reactions. Phase I transformation introduces a polar group (e.g., OH– and NH2–) to the parent drug typically via the cytochrome P450 system (CYPs). While such reactions often inactivate the drug, the addition of these functional radicals may result in formation of active metabolites for some drugs, or cause activation of a prodrug. One example of a prodrug is codeine, which is catalyzed by CYP2D6 and O‐demethylated to form morphine. Local codeine O‐demethylation in the central nervous system could explain the analgesic effect of codeine despite relatively low plasma levels of morphine [13]. The CYP450 system is the most important group of metabolizing enzymes in the body. They are found in several tissues, such as the liver, kidney, and intestines, and are responsible for the oxidation and reduction of xenobiotics and endogenous substances. These reactions convert relatively lipophilic compounds into hydrophilic metabolites to facilitate their elimination through the urine or bile. They are mostly carried out intracellularly in the microsomes of the smooth endoplasmic reticulum (i.e., microsomal enzymes). Zone 3 hepatocytes have the highest content of CYP450 enzymes. Their localization is important in predicting the region where hepatic damage will be most evident when a drug is metabolized to toxic substances. An example is severe centrilobular necrosis and cholangiohepatitis observed in some cats following administration of oral diazepam [14]. Although the same families and subfamilies of P450 enzymes are present in most domestic species, different individual enzymes might be encountered in each species. Additionally, the same enzyme may be present but act on a different substrate range compared with humans [15]. Therefore, it becomes problematic to extrapolate drug doses, intervals, effects, and metabolic fate from human‐derived data to veterinary species. Differences in CYP activity exist not only among species but also among different breeds and genders. For example, Beagles have greater propofol hydroxylase activity (CYP2B) than Greyhounds [16]. Gender variability in dogs and cats for different CYP families has also been described [17]. Phase II reactions are responsible for conferring further hydrophilicity to a drug, facilitating its excretion. These reactions occur mostly in the cytosol. The domestic cat has been recognized to have a reduced ability to form glucuronide conjugates of many xenobiotics (e.g., propofol) [18]. Therefore, when low molecular weight phenolic derivatives are given to cats, they are biotransformed slowly, or are biotransformed and eliminated by other mechanisms [19]. Defects of the UDP‐glucuronosyltransferase (UGT)1A6 gene seem to be responsible for the poor glucuronidation of some drugs in cats, such as acetaminophen [20]. However, cats have other UGT enzymes that can biotransform some xenobiotics [21]. Acting as the most diverse catalysts, CYPs contribute to interindividual variations in drug responses, resulting from genetic and epigenetic variants, as well as factors such as gender, age, nutritional status, disease states, and pathophysiological states. The CYPs can be inhibited or induced by concomitant drugs and circulating metabolites, which can influence treatment outcomes through drug–drug interaction, drug–gene interaction, and drug–drug–gene interaction [22]. Some xenobiotics can induce CYP and glucuronosyltransferase activity. Ketamine can induce both enzyme types, contributing to the development of its own tolerance in rats [23]. Reduced protein activity results in decreased removal of certain drugs from brain to blood, and from blood to bile on the hepatocellular surface in humans [24]. This may be related to some adverse effects of ivermectins and morphine‐like derivatives from brain to systemic circulation in MDR1 (ABCB1) mutant dogs [25]. In the last two decades, a large amount of information on the metabolism and interaction of specific drugs in veterinary species has been published [26]. However, further research is warranted to characterize individual CYPs for each species and their substrates. The liver has a large role in host defenses. Of the nonparenchymal cells in the liver, four types of antigen‐presenting cells serve as immunologic gatekeepers. These are Kupffer cells, dendritic cells, stellate cells, and sinusoidal endothelial cells, all responsible for innate immunity. Bacteria and toxins that gain access to the portal circulation are routinely phagocytized and processed by Kupffer cells in the sinusoids [27]. Kupffer cells are also scavengers of inflammatory mediators, appearing to limit the extent of the inflammatory response [28]. However, when an overwhelming amount of endotoxin is present, activated Kupffer cells can produce reductive oxygen radicals and cytokines. These processes allow Kupffer cells to signal hepatocytes and endothelial cells, changing their transcription products, in addition to recruiting circulating neutrophils. This hepatic immunologic response can be responsible for hepatocellular injury seen in some disease conditions. The close interaction between the inflammatory response and coagulation also plays a role in the pathogenesis of hepatic and multiorgan failure. For example, during endotoxemia and hypoperfusion, the activated hepatic cells (Kupffer cells, hepatocytes, and endothelial cells) promote an inflammatory, hypercoagulable state [29]. Whenever a patient’s hepatic function is questioned, a biochemical profile, coagulation panel, and substrate metabolism tests should be requested. However, a reduction of approximately 70–80% of hepatic function must be present before observing abnormalities in biochemical parameters; hence, these disturbances are not sensitive indicators of hepatic dysfunction. Limitations of conventional coagulation tests have led to increased interest in viscoelastic testing systems and their use in global hemostasis assays. Substrate metabolism tests such as serum bilirubin, ammonia, and pre‐ and postprandial bile acids are better indicators of hepatic function than serum liver enzyme concentrations [30]. Hyperbilirubinemia can be caused by prehepatic disease (e.g., hemolysis), primary hepatic disease (e.g., decreased hepatocyte function), and post‐hepatic disease (e.g., extrahepatic biliary obstruction). Hyperbilirubinemia is reported in approximately 50% of dogs with chronic hepatitis (CH) and is a negative prognostic indicator [31]. Measurement of serum conjugated (direct) and unconjugated (indirect) bilirubin to distinguish between prehepatic, hepatic, or post‐hepatic hyperbilirubinemia is not clinically reliable [32]. A study evaluating the sensitivity and specificity of fasting ammonia and serum bile acids for diagnosis of portosystemic shunt (PSS) in dogs and cats found the tests to have high sensitivity and specificity in both species [33]. Indocyanine green and sulfobromophthalein clearance tests are quantitative measures of hepatic function, reflecting blood flow‐dependent clearance, hepatocyte uptake, and biliary excretion. Hepatic clearance of indocyanine green can change in direct proportion to variations in liver blood flow, as seen in isoflurane‐anesthetized dogs where a 63% reduction in CO resulted in a 26% decline in clearance [34]. In human patients, the hepatic metabolism of lidocaine is considered as a dynamic liver function test. It is non‐invasive, easy, and quick to perform. Lidocaine is converted to monoethylglycinexylidide (MEGX) and subsequently to glycinexylidide (GX) by the hepatic cytochrome P450 enzyme system. As MEGX accurately reflects the severity of hepatic dysfunction, it is valuable for quantitative evaluation of liver dysfunction along with being a prognostic predictor of liver disease in adults [35]. In dogs, MEGX testing has been shown to successfully differentiate closed extrahepatic PSS from persistent shunting after gradual attenuation surgery [36]. Hepatobiliary disease also results in decreased protein synthesis as well as altered glucose, urea nitrogen, and lipid metabolism. Because the liver normally produces albumin at one‐third of its capacity, hypoalbuminemia is seen only in severe cases of hepatic dysfunction or severe protein loss [32]. Glomerular disease, protein‐losing enteropathies, and hemorrhage are non‐hepatic causes and should be ruled out when hypoalbuminemia is present. Alpha and beta globulins may also be diminished with hepatic dysfunction. Reduction in plasma protein concentrations will decrease the bound fraction of some drugs, which can result in changes in volume of distribution, plasma concentration, and drug effect. Patients with PSS or acute hepatic failure are often hypoglycemic, or at risk for clinical hypoglycemia during the perianesthetic period. Mechanisms of hypoglycemia in hepatic dysfunction include one or a combination of the following: (1) decreased gluconeogenesis, (2) decreased glycogen storage, and (3) diminished response to glucagon. Monitoring and maintenance of normoglycemia should be a priority during anesthesia. Serum cholesterol concentrations can be increased, normal, or decreased in patients with hepatobiliary disease. A recent canine study identified a significant positive association between hyperlipidemia (specifically hypercholesterolemia), liver enzymes, and total serum bile acid (TSBA) concentrations in dogs suffering from CH [37]. Decreased concentrations of blood urea nitrogen and cholesterol were seen to develop in approximately 40% of dogs with CH, occurring most commonly in those with cirrhosis. Hypoglycemia is rare in CH and is more often associated with acute liver failure. The TSBA concentrations are uniformly increased when portosystemic shunting is present, and their sensitivity for detecting cirrhosis and the presence of an acquired PSS is high. Dogs with cholestasis (i.e., hyperbilirubinemia) associated with hepatic disease will always have increased TSBA concentrations. Hyperammonemia has similar sensitivity for detection of CH or cirrhosis and acquired PSS as TSBA and is somewhat more specific because it is not affected by cholestasis [31]. Enzyme concentration abnormalities are common, but their relation to patient risk is often difficult to interpret. The serum concentration of alanine aminotransferase (ALT), aspartate aminotransferase (AST), alkaline phosphatase (ALP), lactate dehydrogenase (LDH), and γ‐glutamyl transpeptidase (GGT) are the most commonly measured hepatocellular enzymes in biochemical profiles. Hepatobiliary tissue damage can cause cellular leakage, resulting in increased plasma concentration of hepatocellular enzymes. However, this rise does not necessarily indicate the degree of hepatic dysfunction. This is especially true for chronic liver diseases, where reduced hepatocellular numbers secondary to fibrosis may result in near‐normal liver enzyme values in the face of severely compromised function. Additionally, elevation of enzymes may represent cellular insult, but may not correlate with any inability to biotransform drugs used for anesthesia and pain management. In several retrospective canine studies, serum ALT activity has shown reliability during CH screening. However, histopathologic evidence of CH can exist in the absence of increased serum liver enzyme activity. As CH progresses and hepatic parenchyma decreases, ALP and GGT activities increase compared to ALT [31]. Even though ALP can be associated with biliary obstruction or injury, its elevation may originate in other locations such as placenta (during pregnancy) and bone. Diagnostic efficacy of serum ALP and GGT was higher (91%) when used together in dogs with histologically confirmed hepatobiliary disease (e.g., cholestasis) [38]. The position of the liver between the splanchnic and systemic circulation creates the potential for diseases of other organ systems to involve the liver, and consequently cause an increase in circulating liver enzymes. Additionally, several enzymes are found in tissue outside the liver (e.g., AST in skeletal muscle, heart, kidney, pancreas, adipose tissue, and brain) and injury to these tissues may be falsely interpreted as hepatic injury. Elevation of liver enzymes, although highly sensitive for hepatocellular injury, is not specific to liver dysfunction. Coagulation status should be considered in patients with liver disease undergoing a surgical procedure, including laparoscopic liver biopsy. CH patients may show no clinical signs of a coagulopathy but may be hypocoagulable and be at a greater risk of coagulopathy after an insult such as surgery [39]. Hepatobiliary disease can give rise to complex alterations in coagulation. These alterations involve changes in pro‐ and anticoagulants, as well as factors participating in fibrinolysis. The net effect of this is that patients with liver dysfunction can have laboratory and clinical features of both hypo‐ and hypercoagulability. This is well demonstrated in dogs with congenital PSS that can be in a hypercoagulable or hypocoagulable state [40,41]. The concomitant changes in both pro‐ and anti‐hemostatic pathways result in a “rebalanced” hemostatic state in these patients. This new hemostatic balance appears to be much more fragile than the hemostatic balance in healthy individuals, and hence, patients with liver disease can readily experience both hemostasis‐related bleeding and thrombotic events. These insights into the hemostatic balance during liver disease warrant revised recommendations for clinical management of hemostasis [42]. Emerging evidence states that such a “rebalanced” state of coagulation also occurs in dogs with liver disease. Whether canine patients have clinical bleeding or thrombosis depends on factors that shift the coagulation state one way or the other. Hemorrhage can be induced by uremia, infection, hypervolemia, or acidosis, whereas thrombosis is precipitated with aggravated portal hypertension, use of corticosteroids, proinflammatory states, or with simultaneously occurring pro‐thrombotic conditions [43]. Prolongations in prothrombin time (PT) and activated partial thromboplastin time (aPTT) and bleeding from provocative procedures suggest hypocoagulable tendencies. Increases in clot strength, D‐dimers, von Willebrand’s factor, and factor VIII activity; decreases in antithrombin and protein C activity; and an increased incidence of thrombosis in the splanchnic circulation suggest hypercoagulable tendencies [44,45]. Fibrinogen also plays several key roles in maintaining both primary and secondary hemostasis and is converted by thrombin into an insoluble fibrin network that, together with platelet aggregates, induces hemostasis in response to rupture of the endothelium. In humans and dogs, plasma fibrinogen concentration during liver cirrhosis progressively decreased with worsening disease, indicating a close relationship between the severity of cirrhosis and hemostatic changes [46,47]. Thrombocytopenia can develop with hepatic disease and is due to mechanisms including decreased platelet production due to thrombopoietin deficiency and direct megakaryocyte toxicity, decreased platelet half‐life due to splenomegaly and hypersplenism, and possibly autoantibodies. Consumption of platelets by intrahepatic or systemic platelet activation may also contribute to decreased platelet counts [42]. In addition, vitamin K malabsorption may develop secondary to certain hepatic diseases (e.g., hepatic lipidosis) or cholestasis (e.g., complete biliary obstruction). Vitamin K acts as a cofactor for the enzyme γ‐glutamyl carboxylase, which activates coagulation factors II (prothrombin), VII, IX, and X. Hence, hepatobiliary diseases can result in secondary hemostasis defects through decreased vitamin K‐mediated carboxylation of coagulation factors [48]. Vitamin K supplementation may be recommended for some liver diseases and could be administered prior to invasive procedures such as liver biopsy or feeding tube placement, due to increased risk of bleeding. Blood typing and crossmatching may be warranted if the coagulation profile is abnormal or if a blood transfusion is anticipated. Fresh frozen plasma (FFP) or cryoprecipitate may be transfused in liver diseases both therapeutically and prophylactically to correct deranged coagulation. However, as the etiology of coagulation defects in liver disease is highly complex, the clinical benefit of prophylactic administration of FFP on the basis of abnormal coagulation tests alone, is questionable [49]. The constituents of FFP include all coagulation factors that are essential to clot formation (i.e., factors II, V, VII, VIII, IX, X, and XI). Repletion of coagulation factors with FFP bypasses the need for vitamin K carboxylation in the liver for coagulation factor formation. In spite of these benefits, FFP transfusion comes with the risk of increasing portal hypertension, hypervolemia, and assorted other risks, including immune‐mediated reactions. Risk of exacerbating portal hypertension and transfusion‐related lung injury argues against routine use of FFP in this population [50]. Other than the conventional coagulation tests, viscoelastic testing systems (VETs) for clot detection are increasingly being used in veterinary medicine for diagnosis of the hemostatic states: normocoagulable, hypercoagulable, hypocoagulable, excessive fibrinolysis, or decreased fibrinolysis. These methods can also evaluate platelet inhibition, functional fibrinogen measurement, and fibrinolysis [51]. Because VETs assess cellular and protein components of hemostasis (platelets and coagulation factors), as well as fibrinolysis, they are referred to as the “global assays of hemostasis.” The VETs provide (1) graphical and numerical results that reflect the dynamic nature of fibrin formation and breakdown, and (2) information on the time of onset of fibrin formation, rate of fibrin formation, strength of fibrin clot, and fibrinolysis at defined intervals. Currently, commercially available VETs include the Sonoclot® Coagulation and Platelet Function Analyzer, thromboelastography (TEG), and rotational thromboelastometry. A novel portable handheld analyzer, the Viscoelastic Coagulation Monitor (VCM Vet™) has been recently introduced for use in dogs and cats to fulfill the clinical need for bedside testing in emergency suites, intensive care units, operating rooms, and field examinations [52,53]. In patients suffering from hepatic disease, VETs may be a superior tool to pinpoint coagulation abnormalities, monitor hemostasis during resuscitation of clinical bleeding disorders, evaluate anticoagulant use, and optimize treatment plans. The diagnostic utility of TEG has been recognized in dogs suffering from acute liver disease, gallbladder mucocele, congenital PSS, and chronic hepatopathies, as well as in cats with cholestatic liver disease [41,43,45,54,55].
40
Physiology, Pathophysiology, and Anesthetic Management of Patients with Hepatic Disease
Introduction
Functional anatomy and physiology
Structural organization of the liver
Hepatic blood flow
Hepatic functions
Protein homeostasis
Metabolic
Synthetic
Homeostatic
Xenobiotic metabolism
Protein metabolism (ammonia, urea)
Lipid metabolism (triglyceride)
Glucose metabolism (gluconeogenesis, glycogenolysis, and glycogenesis)
Carbohydrate metabolism
Nutrient metabolism (amino acids, lipids, and vitamins)
Bile production and secretion
Biotransformation and biliary excretion
Coagulation and anticoagulation proteins
Plasma proteins (albumin, alpha‐1 acid glycoprotein)
Steroid hormones (cholesterol)
Thrombopoietin
Angiotensinogen
Insulin‐like growth factor
Fetal and extramedullary hematopoiesis
Acute phase proteins
Plasma cholinesterases
Intravascular volume regulation (RAAS)
Glucose homeostasis
Regulation of portal blood flow (HABR)
Liver regeneration and growth
Plasma oncotic pressure
Innate and adaptive immunity
Coagulation
Biotransformation and elimination of xenobiotics
Immune and inflammatory response
Assessment of hepatic function
Biochemical derangements in liver pathology
Interpretation of liver enzymes
Assessment of coagulation
Anesthetics and hepatic disease
Sedatives
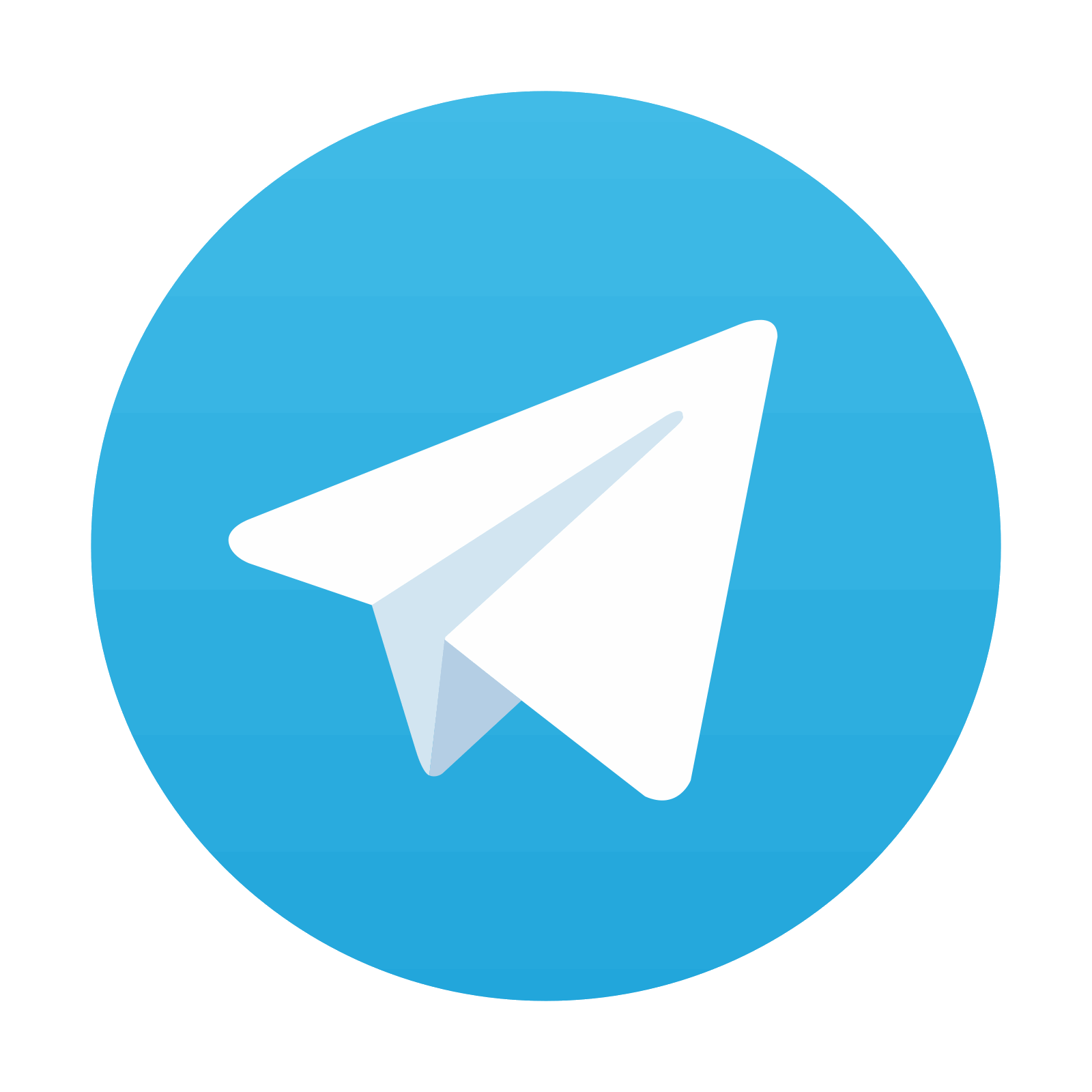
Stay updated, free articles. Join our Telegram channel
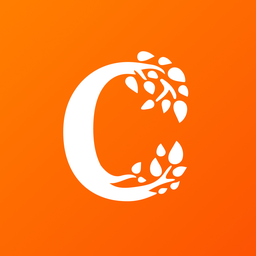
Full access? Get Clinical Tree
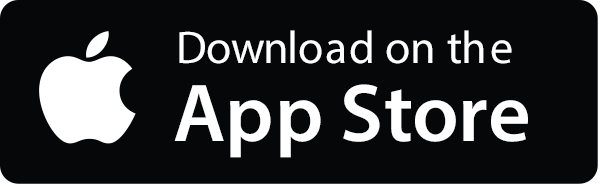
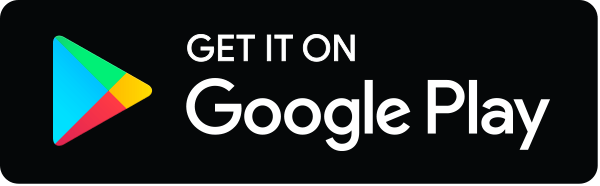