The horse karyotype
Ever since the correct chromosome number in the horse was determined, the equine chromosomes have been presented in several ways. However, it was not before the correct number of metacentric and sub-metacentric (13 pairs of autosomes+the sex chromosomes) and acrocentric (18 pairs of autosomes) chromosomes was ascertained (Benirschke et al., 1965; Hsu and Benirschke, 1967) that a stable karyotype pattern started to emerge. This led to the development of the first (Ford et al., 1980) and the second (Richer et al., 1990) karyotype standards for the horse (for details, see Chowdhary and Raudsepp, 2000). Despite being useful for cytogenetic analysis, the standards fell short of an enumerated nomenclature of chromosome bands and were of limited use for gene mapping. To cater to the needs of both cytogenetics and gene mapping in correctly identifying equine chromosomes, a new high-resolution international system of chromosome nomenclature for the horse was developed (ISCNH, 1997). The new nomenclature provided a detailed description of both G- and R-banded chromosomes along with idiograms showing landmarks and band numbers for each of the banding approaches (Figure 3.1). Since then, the ISCNH 1997 has served as the internationally accepted platform for equine clinical cytogenetics and physical gene mapping.
Figure 3.1 G-banded horse standard ideogram (ISCNH, 1997). Segmental homology with human chromosomes is shown to the right of each horse chromosome (Raudsepp et al., 1996; Raudsepp et al., 2008; Yang et al., 2004b).

Gene Mapping in Horses – Historical Background
Gene mapping studies in horses date back almost five decades, when G6PD was assigned to the X-chromosome (Mathai et al., 1966; Trujillo et al., 1965). In 1970s–1980s, significant contributions were made to develop equine linkage maps (Andersson and Sandberg, 1984; Sandberg, 1974; Sandberg and Andersson, 1984), but chromosome and karyotype studies in the horse had not progressed sufficiently to physically assign any of the linkage groups to specific chromosomes. With the exception of cytogenetic localization of rRNA gene clusters to autosomes in late 1970s (Sysa et al., 1977), the formal beginning of physical gene mapping in horses falls into late 1980s when the major histocompatibility complex (ELA) was assigned to ECA20 by radioactive in situ hybridization (Ansari et al., 1988; Makinen et al., 1989). The coming years showed a slow but steady progress in gene mapping. The main stimulus came in 1995 when the international equine community, under the aegis of the Dorothy Russell Havemeyer Foundation, decided to use a concerted approach to map the horse genome. This was possible because both the breeders and the horse geneticists agreed that a framework map is necessary to study the underlying genetics of numerous congenital disorders known in horses and to find means to control them. Since then, FISH and radiation hybrid panels have occupied the central role in constructing physical maps. Development of resources, such as cDNA and bacterial artificial chromosome (BAC) libraries (for review, see Rubes et al., 2009), and identification of microsatellite and gene-specific markers (Godard et al., 1998; Godard et al., 1997; Guérin et al., 1999; Lindgren et al., 1998; Milenkovic et al., 2002; Tozaki et al., 2007) have provided tools for the construction of cytogenetic (Milenkovic et al., 2002), radiation hybrid (Chowdhary et al., 2003; Raudsepp et al., 2008) and high-resolution BAC contig maps (Brinkmeyer-Langford et al., 2008; Gustafson et al., 2003; Raudsepp and Chowdhary, 2008b). Altogether, more than 5,000 loci have been mapped in the horse genome using various physical mapping approaches (Paria, 2009; Raudsepp et al., 2008). Of these, about 900 loci integrate physical and genetic linkage maps, while about 2,000 loci are aligned with the human genome sequence and establish a comparative framework between the two genomes (Raudsepp et al., 2008). Most importantly, integrated physical, meiotic, and comparative maps have been instrumental for the assembly and annotation of the horse genome sequence (Wade et al., 2009).
Cytogenetic Map
Fluorescence in situ hybridization
Fluoresence in situ hybridization (FISH) to nuclear chromatin is the most direct approach for constructing cytogenetic maps. The method relies on Watson-Crick base pairing complementarity principle and permits the location of DNA markers in their original place, or in situ (Raudsepp and Chowdhary, 2008a). The two major components of ISH are the probe and the target. In case of cytogenetic maps, the targets can be mitotic or meiotic chromosome preparations at different stages of the cell cycle, DNA fibers (Raudsepp and Chowdhary, 2008a; Rubes et al., 2009; Speicher and Carter, 2005; Trask, 2002), or DNA tiling-arrays (Shaffer and Bejjani, 2006; Sharp, 2009). The probes vary considerably in size and origin, from a few-base-pairs-long telomeric or centromeric repeats to composite DNA sequences from the whole chromosome, chromosomal segment, or the whole genome. The probes are labeled directly with fluorochromes or indirectly with molecules that need binding by fluorochrome-conjugated antibodies to visualize the signals. Over the past 25 years, FISH methodology has improved tremendously (Dobigny and Yang, 2008; Lichter, 1997; Nederlof et al., 1989; Rubes et al., 2009; Speicher and Carter, 2005; Trask, 2002). Sophisticated imaging systems and the available number of spectrally distinct fluorochromes allow simultaneous detection of two, three or even multiple probes, and the overall sensitivity of FISH has increased about 10,000 times.
FISH mapping in the horse
Direct physical assignment of loci to horse chromosomes by in situ hybridization started more than twenty years ago using the radioactive approach. Due to the lack of horse-specific probes, human and pig genomic or cDNA clones were used. The first in situ mapped loci were the equine major histocompatibility complex (ELA) to ECA20q14-q22 (Ansari et al., 1988; Makinen et al., 1989) and glucosephosphate isomerase (GPI) to ECA10pter (Harbitz et al., 1990). The fluoresence method was for the first time used to assign hemoglobin alpha (HBA) to ECA13q (Oakenfull et al., 1993). From that point on, FISH has been the primary approach for the construction of cytogenetic maps in the horse.
During the early days of FISH mapping, the main limitation was the availability of suitable probes. Clones containing equine genes and microsatellites were PCR products, short cDNA sequences, or originated from relatively small-insert phage and cosmid libraries (Breen et al., 1997b; Godard et al., 1998; Godard et al., 2000; Godard et al., 1997). Sometimes, due to the lack of suitable horse probes, heterologous human or porcine BACs were used for mapping (Raudsepp et al., 1999). Despite this, in less than seven years, more than 331 DNA markers and 48 genes/ESTs were localized to equine chromosomes by FISH (for review, see Chowdhary and Raudsepp, 2000). An important breakthrough in cytogenetic mapping came with the availability of the INRA (Godard et al., 1998; Milenkovic et al., 2002), CHORI-241 (http://bacpac.chori.org/equine241.htm), and TAMU (http://hbz7.tamu.edu/homelinks/bac_est/bac.htm) large-insert genomic BAC libraries (for review, see Rubes et al., 2009). The use of BAC clones, which contain 150–200 kb fragments of the horse genome, essentially improved the quality of hybridization signals and led to rapid qualitative and quantitative improvements in FISH mapping. As a result, the number of FISH mapped markers in the horse genome started to increase rapidly. Majority of publications reported FISH assignment of a single gene or a small number of loci (e.g., (Breen et al., 1997b; Lear et al., 1998a; Lear et al., 1998b; Lear et al., 2000; Raudsepp et al., 1999; Raudsepp et al., 1997), while more comprehensive studies involved about 30–50 loci (Godard et al., 1998; Godard et al., 2000; Godard et al., 1997; Lear et al., 2001; Lindgren et al., 2001; Mariat et al., 2001; Perrocheau et al., 2005). A few studies consolidated all available map information and constructed FISH maps for all autosomes and the X chromosome (Chowdhary et al., 2003; Milenkovic et al., 2002; Perrocheau et al., 2006; Raudsepp et al., 2008). Cytogenetic mapping was also used to localize candidate genes for several equine conditions, such as recurrent airway obstruction (Jost et al., 2007; Klukowska-Rotzler et al., 2006a), genes involved in circadian clock (Murphy et al., 2007), infection (Horin et al., 2008), immunity (Musilova et al. 2005), and the development of skeletal, gastrointestinal, cardiovascular, and nervous systems (Zabek et al., 2009; Zabek et al., 2007). Also, FISH has been instrumental for validating the assembly of high-resolution BAC contig maps that were constructed for the ELA (Gustafson et al., 2003), a 3.3 Mb region on ECA21 (Brinkmeyer-Langford et al., 2008), the pseudoautosomal region (Raudsepp and Chowdhary, 2008b), and the Y chromosome (Paria, 2009; Raudsepp et al., 2004b). Likewise, FISH has been extremely useful in accurate physical alignment and orientation of synteny, genetic linkage, and RH maps (Brinkmeyer-Langford et al., 2005; Chowdhary et al., 2002b; Chowdhary et al., 2003; Goh et al., 2007; Gustafson-Seabury et al., 2005; Lee et al., 2004; Perrocheau et al., 2006; Raudsepp et al., 2008; Wagner et al., 2006), as well as for the draft assembly of the genome sequence (Lear et al., 2007; Wade et al., 2009). Presently, 1,144 loci (Raudsepp et al., 2008) have been assigned by FISH to equine chromosomes (Table 3.1). Given that the estimated size of the horse genome is 2500–2700 Mb (Wade et al., 2009), this provides an average of one cytogenetically mapped marker at every 2.2–2.4 Mb of the genome.
Multicolor and high-resolution FISH
Horse is probably the only domestic species where both multicolor and high-resolution FISH have been extensively used in physical gene mapping. Dual- or triple-color FISH on metaphase, prometaphase, and interphase chromosomes (Figure 3.2) have been pivotal in orienting and ordering closely located markers, particularly markers that are tightly linked in the RH and meiotic maps.
Figure 3.2 Multicolor FISH: (a) dual-color FISH to order two genes on ECAX in metaphase; (b) three-color FISH to order three closely located loci on ECA27 in interphase.

An essential improvement in FISH resolution is provided by fiber-FISH. Typically, DNA probes are mapped to metaphase chromosomes at a resolution of about 2–5 Mb (Raudsepp and Chowdhary, 2008a). Fiber-FISH, on the other hand, enables to distinguish probes separated by 1–2 kb and can be used for positional cloning, determining transcriptional orientation of clones, or detection of minor chromosomal rearrangements (Laan et al., 1996). In the horse, the technique has been used for validating contigs of overlapping BAC clones in the pseudoautosomal region (Raudsepp and Chowdhary, 2008b) and the male-specific region of the horse Y chromosome (Raudsepp et al., 2004b; see also Chapter 5).
Physical mapping using flow-sorted and microdissected chromosomes
Another source of probes for cytogenetic mapping is flow-sorted and microdissected chromosomes. In flow sorting, a fluorescence activated cell sorter system (FACS) separates individual chromosomes based on their DNA content and the relative proportion of AT/GC sequences (Lebo, 1982; Lebo and Bastian, 1982). The procedure results in generating a flow karyotype, distinguishing each chromosome (Lebo, 1982). Once separated, collected, and amplified, DNA from individual chromosomes is available for various mapping purposes. In contrast to other domestic species where flow karyotypes were generated in late 1990s (see Chowdhary and Raudsepp, 2005), flow sorting of horse chromosomes had a late start. The only flow karyotype of the horse was generated in 2004 and used for comparative chromosome painting to human, donkey, and mule chromosomes (Yang et al., 2004a), and later for karyotype comparisons with other equids and Perssiodactyls (Trifonov et al., 2008). Additionally, flow-sorted ECAX has been used to detect X chromosome aneuploidies (Breen et al., 1997a). Notably, ECAY is the only flow-sorted horse chromosome that has been used for physical mapping and gene discovery (Paria, 2009; Paria et al., 2011; see Chapter 5).
A convenient alternative to flow sorting is chromosome microdissection, which allows isolation of DNA from whole chromosomes, as well as from chromosome arms, segments, or bands (Chaudhary et al., 1998; Chowdhary and Raudsepp, 2001). The technique involves manual (Guan et al., 1992; Meltzer et al., 1992) or laser-assisted (Kubickova et al., 2002) microscopic scraping of chromosomal regions of interest, followed by microdissected DNA amplification by DOP-PCR (Telenius et al., 1992), or some other whole genome amplification method (Sorensen et al., 2007). The amplified DNA is labeled and used as a painting probe for FISH experiments or as a source of DNA for cloning and mapping.
In horses, microdissected probes were first generated for all metacentric and sub-metacentric autosomes and the sex chromosomes (Raudsepp and Chowdhary, 1999), and later for all chromosomes (Bugno et al., 2009a). Additionally, arm-specific probes are available for the X chromosome (Bugno and Slota, 2007) and bi-armed autosomes (Musilova et al., 2007). These composite probes have been used for cross-species chromosome painting between horse and donkey (Raudsepp and Chowdhary, 1999) and for molecular cytogenetic analysis of various chromosomal abnormalities (Bugno and Slota, 2007; Bugno et al., 2007b, 2009a). In a few cases, chromosome-specific probes have found use for physical gene mapping. For example, microdissected ECA1 (Bowling et al., 1998) and ECA6 and ECA12 (Chowdhary et al., 1998b) were used to construct chromosome specific libraries and identify microsatellite markers. Some of these markers, namely 1CA01-44, RKJ6, and RKJ12, have been mapped to the corresponding chromosomes by FISH and/or RH analysis (Raudsepp et al., 2008).
FISH for mapping chromosome and genome rearrangements
Molecular hybridization-based methods, initially developed for physical gene mapping, have become an integral part of equine clinical cytogenetics (Durkin et al., 2010; Lear and Bailey, 2008; Rubes et al., 2009; see also Chapters 16 and 18). Molecular hybridization with chromosome-specific paints is not only an efficient method for detecting aneuploidies in chromosome preparations (Breen et al., 1997a; Bugno and Slota, 2007; Bugno et al., 2007a; Bugno et al., 2007b), but also in sperm (Bugno-Poniewierska et al., 2009) and embryos (Rambags et al., 2005). Horse BAC clones containing known genes or markers are ideal tools for detailed analysis of complex structural rearrangements (Durkin et al., 2008, 2010; Lear and Bailey, 2008; Lear et al., 2008) and for identification of chromosomes involved in autosomal trisomies (Brito et al., 2008).
Recently, as a joint effort of researchers at Texas A&M University (United States) and the University of Adelaide (Australia), and building on the available genome sequence assembly (Wade et al., 2009), a whole genome oligonucleotide tiling array was constructed for the horse (Qu et al., 2011). This next-generation research tool uses comparative genomic hybridization (CGH) as the analysis method, and is therefore also known as a CGH array. The equine tiling array comprises of 418,577 probes that are tiled into repeat-masked EquCab2 with an average resolution of 7.5 Kb. The array contains a subset of 82,354 probes representing exons of almost all 18,763 annotated equine genes. The Y chromosome is represented by 519 probes, and 26,790 probes originate from 3 Mb long sub-telomeric regions of all autosomes. This CGH array is the first comprehensive equine high-resolution resource for mapping copy number variants (CNVs) and other large-scale submicroscopic structural rearrangements (Pang et al., 2010; Shaffer and Bejjani, 2006; Sharp, 2009). It is believed that array CGH will help uncover the extent of naturally occurring structural variations in horse populations, and will also lead to the discovery of regions associated with complex traits and genetic disorders. Regardless of the specific goals, array CGH analysis of the horse genome will provide new applications to FISH although any significant copy number gain or loss needs validation by FISH on metaphase or interphase chromosomes (Liu et al., 2010).
With the whole genome sequence available (Wade et al., 2009), the practical need for FISH mapping in the horse is rapidly decreasing. For example, in 2006–2007, around a dozen publications reported FISH assignment of about 30 new loci; by 2008–2009, the number of publications had decreased to less than 10, and in 2010, there were no reports on cytogenetic mapping in the horse. Instead, equine BACs are finding use as probes for FISH mapping in the donkey (Bugno-Poniewierska et al., 2010; Bugno et al., 2009b) and other equids (Piras et al., 2009). Thus, the golden years of FISH mapping of the equine genome are irreversibly over. However, this elegant, precise, and visually attractive mapping approach will continue to carry out important tasks in equine genome analysis. FISH will be needed to complete the horse genome assembly and physically anchor the 5% of genome sequences that are currently unassigned (Wade et al., 2009). Also, FISH and equine BAC clones remain valuable tools for the detection of various genomic rearrangements, both at chromosomal and submicroscopic levels.
Somatic Cell Hybrid (SCH) Panels and Synteny Mapping
Somatic cell genetics (Faraut et al., 2009; Kao, 1983; Kucherlapati and Ruddle, 1975; Ruddle, 1981) has been the key to the development of hybrid cell panels commonly used for generating synteny maps. Over the years, very little has changed in terms of the approaches used to develop these panels in different species.
The first syntenic group identified in the horse comprised of three X-linked genes, namely G6PD, HGPRT and PGK, that were indirectly mapped by analyzing a mule x mouse hybrid cell panel (Deys, 1972). After that, four more SCH panels were constructed (Bailey et al., 1995; Lear et al., 1992; Raney et al., 1998; Shiue et al., 1999; Williams et al., 1993). Of these, only one panel was characterized using FISH with equine genomic DNA (Lear et al., 1992). By 1995, 9 syntenic groups with 25 markers were established in the horse using enzymatic analysis of lysates (Williams et al., 1993) or PCR (Bailey et al., 1995). None of the groups could be assigned to specific chromosomes. Later, the panel created at UC Davis (Shiue et al., 1999) became the main contributor to the equine synteny map. With around 450 markers analyzed on this panel, the resource was central in assigning synteny groups (comprising genes and microsatellites) to individual equine autosomes (Caetano et al., 1999a; Caetano et al., 1999b; Lindgren et al., 2001; Shiue et al., 1999) and the sex chromosomes (Shiue et al., 2000). The resource contributed also to the development of the horse-human comparative map (Caetano et al., 1999c; Chowdhary et al., 2003). The use of SCH panels and synteny mapping in the horse came to an end in mid-2000s. Part of this was attributed to depleting DNA reserves from the original panel, though the main reason was the availability of other more advanced mapping approaches.
Radiation Hybrid (RH) Panels and RH Mapping
RH mapping methodology
Radiation hybrid (RH) mapping is essentially a SCH technique with the difference that before fusion of cell lines, the cells of the species of interest (donor) are exposed to high doses of X-ray irradiation that cause fragmentation of chromosomes (Chowdhary and Raudsepp, 2005; Faraut et al., 2009). The dosage may range from as low as 3,000 rad (Gyapay et al., 1996) to as high as 50,000 rad (Lunetta et al., 1996). The higher is the radiation dose, the higher is the resolution and mapping power of the RH panel because more breaks and smaller chromosomal fragments are produced. Higher radiation doses also mean that more markers need to be genotyped in order to construct RH maps. It has been calculated that in a typical mammalian genome, an irradiation dose of 3,000 rad should allow mapping of about 3,000 markers, and about 12,000 markers can be mapped to unique positions on a 12,000 rad RH panel (Chowdhary and Raudsepp, 2005; Faraut et al., 2009). Traditionally, RH genotyping is carried out by PCR, and any type of markers, regardless of their polymorphism status, can be assigned to RH maps. The data are analyzed with dedicated software programs, of which CONCORDE (Agarwala et al., 2000) and CARTHAGENE (de Givry et al., 2005) have been recently used most.
Horse RH panels
Two horse x hamster radiation hybrid panels – a 3,000 rad (Kiguwa et al., 2000) and a 5,000 rad (Chowdhary et al., 2002a) – have been made in the horse. The 3,000 rad panel with 94 hybrid clones provided preliminary maps for horse chromosomes 1 and 10, and since then has not been used for additional analysis. Contrary to this, the 5,000 rad panel with 93 hybrid clones has been the sole resource for constructing chromosome specific and whole genome RH maps and for rapid assignment of loci of interest to chromosomes or ordering them in relation to mapped markers.
RH mapping in the horse
The 5,000 rad panel was first used to obtain low-resolution RH and comparative maps for ECA11 (Chowdhary et al., 2002b) and ECAX (Raudsepp et al., 2002). Once the two studies had demonstrated the high utility of the panel for mapping the horse genome, systematic genotyping of all available, as well as newly generated, Type I and Type II markers was initiated worldwide. These joint efforts led to the construction of the first-generation medium-density RH and comparative map of the horse genome (Chowdhary et al., 2003). The map incorporated 730 markers (258 Type I and 472 Type II) and, for the first time, integrated synteny, cytogenetic, and meiotic maps into a comprehensive consensus map for all autosomes and the X chromosome.
Since then, the 5,000 rad RH panel has been one of the primary tools for mapping the horse genome. Targeted generation of gene-specific and polymorphic markers led to the construction of high-resolution (on average 1 marker/Mb) RH and comparative maps for individual chromosomes, namely ECA17 (Lee et al., 2004), ECAX (Raudsepp et al., 2004a), ECAY (Raudsepp et al., 2004b), ECA22 (Gustafson-Seabury et al., 2005), ECA15 and ECA18 (Wagner et al., 2006), parts of ECA7, ECA10, and ECA21 (Brinkmeyer-Langford et al., 2005), and ECA14 and ECA21 (Goh et al., 2007) or chromosomal regions, specifically ECA4q (Dierks et al., 2006). Concurrently, a medium-density map of the horse genome was reported by adding 165 gene-specific markers to the existing maps (Perrocheau et al., 2006). Additionally, several research groups worldwide have used the RH panel to independently map a number of gene-specific and microsatellite markers (e.g., Berg et al., 2008; Boneker et al., 2005a; Boneker et al., 2005b; Brenig et al., 2004; Bricker et al., 2005; Klukowska-Rotzler et al., 2006a; Klukowska-Rotzler et al., 2006b; Leeb et al., 2005; Mickelson et al., 2004; Momozawa et al., 2005, 2006, 2007a; Muller et al., 2005a,b,c; Prause et al., 2007; Takahashi et al., 2004; Wagner et al., 2004a; Wagner et al., 2004b; Wagner et al., 2004c; Wittwer et al., 2005). In 2008, the concerted international mapping efforts resulted in the construction of a second-generation whole-genome RH and comparative map for the horse genome (Raudsepp et al., 2008). This high-resolution map consists of 4,101 markers distributed over all autosomes and the X chromosome (http://content.karger.com/ProdukteDB/miscArchiv/000/151/313/Fig1.pdf). The overall resolution of the map is one marker every 720 kilobases, which represents a 6-fold improvement over the first-generation map. The map integrates available genetic linkage (907 RH mapped markers are shared with the AHT and IHRFP linkage maps; see Chapter 2) and RH mapping data into a physically ordered map for all equine chromosomes, except ECAY (Figure 3.3). The assignment and orientation of various linkage groups is strongly supported by 1,144 FISH-mapped markers. The integration of the RH and the two most recent meiotic maps (Figure 3.4) has been useful in getting a comparative overview of the three maps, particularly because the linkage maps were obtained using different reference family resources, and in some instances show disagreements (http://content.karger.com/ProdukteDB/miscArchiv/000/151/313/Fig2.pdf). This high-resolution map has been a valuable tool for the identification of genes and/or markers associated with economically important traits like disease resistance or susceptibility, growth, reproduction, and performance. The RH panel has been used to map candidate genes for equine conditions, such as recurrent airway obstruction (Klukowska-Rotzler et al., 2006a), glycogen storage disease IV (Ward et al., 2003), and even temperament and anxiety (Momozawa et al., 2006; Momozawa et al., 2007b). Besides, the integrated radiation hybrid map (Raudsepp et al., 2008) has been instrumental for anchoring and orienting whole genome sequence contigs and scaffolds on horse chromosomes (Lear et al., 2007; Wade et al., 2009). Last but not least, it is noteworthy that more than 12.5% of all currently known protein coding genes in the horse genome (Table 3.1) have been mapped using a combination of RH and other physical mapping approaches.
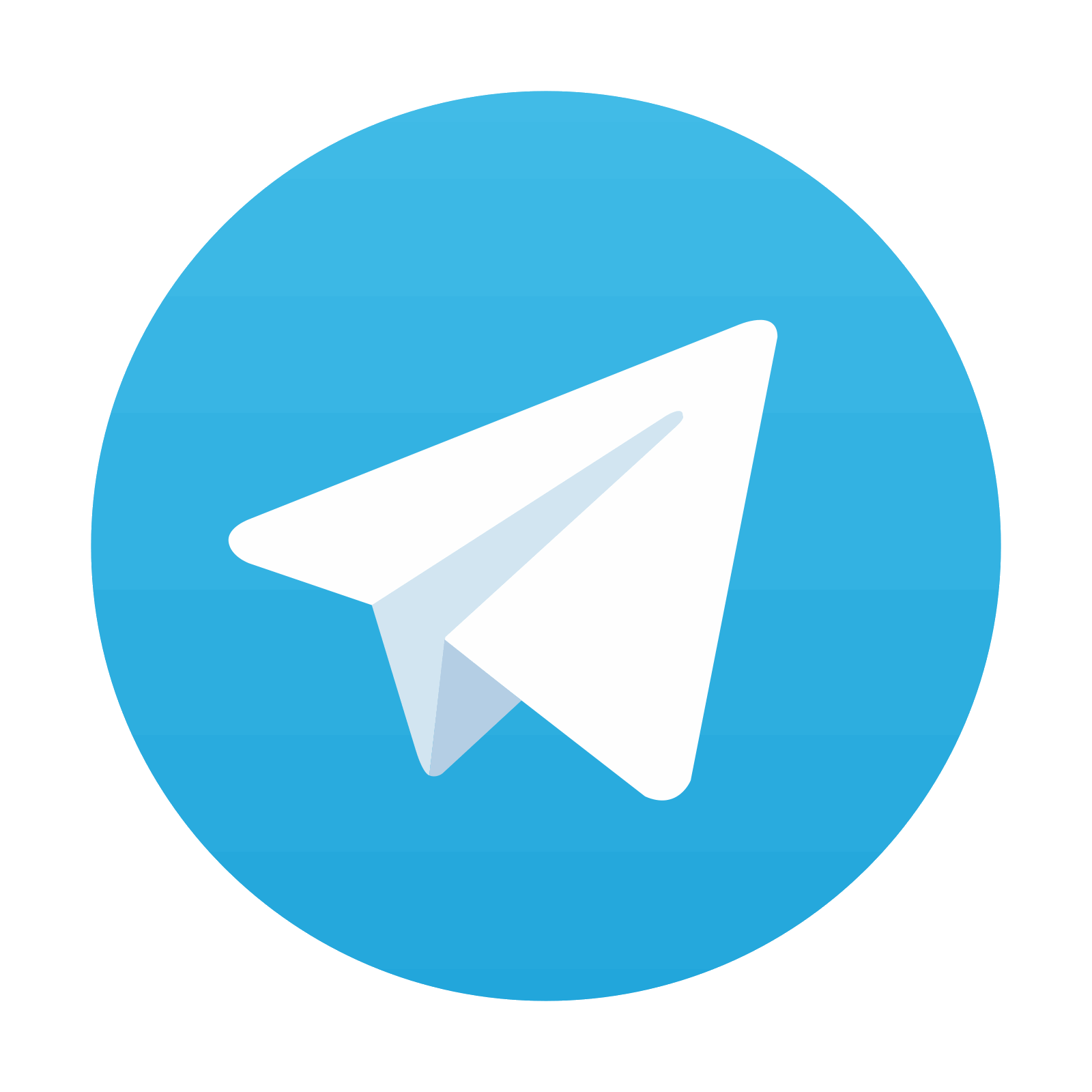
Stay updated, free articles. Join our Telegram channel
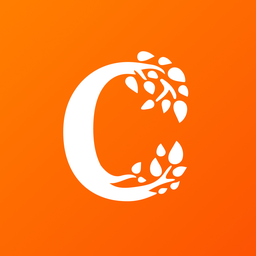
Full access? Get Clinical Tree
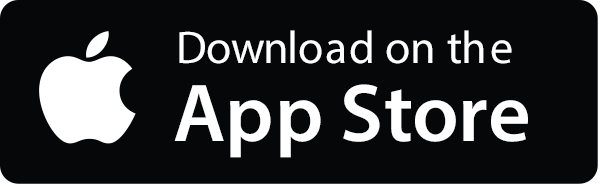
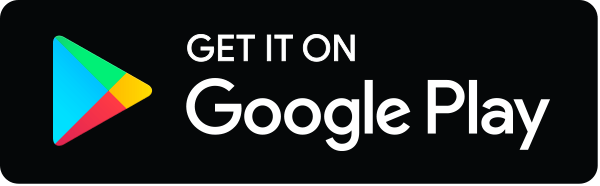