Application of different banding techniques
A variety of staining/banding techniques have been applied to analyze equine chromosomes. The ultimate goal of using these banding techniques was to exploit a range of structural and functional features that permit unambiguous discrimination of individual pairs of homologous chromosomes. The dyes used to stain the chromosomes can be fluorescent or nonfluorescent. The most common staining technique for visualization of chromosomes is the use of Giemsa (Trujillo et al., 1962). The Q-banding (Caspersson et al., 1970) and G-banding (Seabright, 1971) techniques highlight AT-rich DNA as positive bands. The R-banding technique (positive bands are reverse to the G- and Q-positive bands) primarily highlights GC-rich DNA by fluorescent (RBG; Molteni et al., 1982) as well as heat treatment/Giemsa-staining-based approaches (RHG; e.g., Power, 1987). These bands can be enhanced by obtaining elongated chromosomes via using cell cycle synchronization and incorporation of bromodeoxyuridine (BrdU) into chromosomal DNA (e.g., Romagnano et al., 1983; Marki and Osterhoff, 1983; Power, 1987a, 1990) as done by Rønne et al. (1993). Essentially, bands are like bar codes that are unique for a chromosome pair among the pairs of homologous chromosomes in a species, which allows distinguishing the homologues from the set of chromosomes in a metaphase spread.
Some banding techniques like the C-banding specifically depict constitutive heterochromatin. The “bands” or regions are highlighted by acidic and/or alkaline and heat treatment, and are seen on almost all horse chromosomes, except ECA11. Interstitial heterochromatin is reported on ECA1pter, 12q, and Xq (most prominent), whereas the Y-chromosome is known to be largely heterochromatic (Figure 1.2). T-banding, which highlights telomeric repeat sequences present at their usual terminal location of the chromosome with no intercalary presence, is observed in horses. They were first reported using molecular cytogenetic approaches (de La Seña et al., 1995). Next, regions containing transcriptionally active ribosomal RNA genes (rDNA or nucleolus organizing regions, NORs) are visualized by NOR-banding carried out by staining chromosome preparations with silver nitrate (Goodpasture and Bloom, 1975). Molecular approaches allow identification of both active and inactive regions in a cell. Typically, ECA1, 27, 28, and 31 are considered as the NOR-bearing chromosomes. In addition to these banding techniques, attempts have also been made to produce bands on equine chromosomes specific for electron microscopy (Messier et al., 1989; Richer et al., 1989), which has even allowed the construction of a karyotype.
Figure 1.2 Two C-banded metaphase spreads showing distinct heterochromatic band at the centromere of most chromosomes. Additional sites (intercalary) are apparent on the long arm of the X chromosome (arrows). Left: a normal female horse (2n = 64XX); right: a female horse with X chromosome trisomy (2n = 65XXX).

Chromosome aberrations – a brief overview
A vast array of reports on chromosomal abnormalities shows that equine chromosomes have been fairly extensively studied, even though the number of published reports is substantially lower than in cattle and pigs. As in other mammals, these chromosomal aberrations are classified into two major categories, namely (1) autosomal (includes autosome-sex chromosome and vice versa translocations) and (2) those involving only the sex chromosomes. Each of the categories is further classified into two subheads: structural and numerical. A large number of mosaic/chimeric karyotypes involving the sex chromosomes, as well as cases of sex-reversal, have been reported. We estimate that not more than 3,000 horses (predominantly mares) have been examined cytogenetically, resulting in the detection of about 400 cases with chromosome aberrations. This count does not include about 120 cases of sex reversal. As the majority of these cases were hand-picked, either due to phenotypic deviations or due to fertility problems, no proportionate evaluation of the frequency of chromosomal aberrations in the horse could be projected.
Rather few structural aberrations have been detected in horses. These include deletions (loss of part of a chromosome), translocations (both balanced and unbalanced), inversion, and duplication. Figure 1.3 shows one of the most recently discovered balanced translocations in the horse that has been analyzed using traditional and molecular techniques (Das et al., forthcoming). All numerical aberrations in horse are trisomies of different autosomes, all of which involve small acrocentric chromosomes (nos. 23–31). Some of the salient features of viable individuals with autosomal aberrations (both structural and numerical) are: reduced to completely impaired fertility, minor to moderate anatomical malformations primarily affecting gait or orientation, atypical poor confirmation, and moderate to notably smaller height as compared to the breed/parents average. Like in other species, production of offspring to full term by individuals bearing balanced reciprocal translocations and tandem fusions has been reported in the horse (see Power, 1991; Long, 1996; Durkin et al., forthcoming; Das et al., forthcoming); still, invariably varying degrees of reduced fertility is observed. However, foaling of an ECA26 trisomy carrier to produce a karyotypically normal colt (Bowling and Millon, 1990) is noteworthy.
Figure 1.3 Heterozygous autosomal translocation between chromosomes 4 and 10 in a mare with a history of recurrent early embryonic loss: (a) distal half of the long arm of chromosome 4 is inverted 180° and translocated to the tip of the short arm of chromosome 10 (red arrows); (b) Fluorescence in situ hybridization (FISH) showing the translocation breakpoint to be in chromosome 4 between markers NPY and UMNe104; (c) Same FISH results shown in interphase (upper) and metaphase chromosomes (lower).

The vast majority of chromosomal abnormalities reported in the horse involve the sex chromosome (predominantly the X). Of the approximately 350 abnormal karyotypes reported to date, more than 90% involve the sex chromosomes. The types of structural chromosome abnormalities involving the sex chromosomes vary from translocations and deletions to isochromosomes, invariably all impacting fertility. A wide range of numerical aberrations of the sex chromosomes are known in the horse, of which monosomy (lack) of the X chromosome (karyotype constitution 63,XO) is the most common. Few cases of pure trisomy of the X chromosome (65,XXX) have also been reported in the horse. Typically, XO and XXX mares are infertile. Next, cases of pure 65,XXY (Kubien et al., 1993) and 66,XXXY (Gluhovschi et al., 1970, 1975) have also been reported.
Finally, various categories of mosaics/chimeras involving the sex chromosomes (primarily the X) have hitherto been reported in horses. It is noteworthy that most of the reported chimeric/mosaic cases are registered as females with a wide range of clinically detectable reproductive system deviations. The animals show varying degree of virilization of the external genitalia.
Sex reversal, most appropriately described as a disagreement between the karyotypic sex and the phenotypic/anatomical sex, has been described in horses. Approximately 135 such cases have to date been observed. Normally, individuals with XY sex chromosome constitution are expected to be males (64,XY). Sex reversal is a condition in which the aforementioned individuals appear more like females, with varying degrees of male-like characteristics. The terminology holds even if the karyotype indicates the animal to be a female (64,XX), but phenotype and clinical examination show the preponderance of male-like characteristics. Some of these cases and associated molecular work we carried out to analyze these cases in relation to the observed micro-deletions on the horse Y chromosome are described in Chapter 5.
Mitochondrial Genome of the Horse
Structure, function, and utility
The mitochondria (mt) are the powerhouses of cells and are responsible for more than 90% of mammalian energy production. All aerobic respiration within cells occurs in this organelle, making it one of the most critical structures for eukaryotes. There are five important protein complexes that are part of the electron transport chain that metabolizes carbohydrates and fatty chain amino acids during production of ATP. The horse mitochondrial genome is ∼16,660 bp in length and includes 13 proteins (NADH1, NADH2, NADH3, NADH4L, NADH4, NADH5, NADH6, COXI, COXII, COXIII, CYTB, ATP6, and ATP8) that are part of 5 protein complexes, 22 transfer RNAs, and 2 ribosomal RNAs (12 rRNA and 16s rRNA; Xu & Arnanson 1994). The mt genome also has a highly variable control region approximately ∼1,192 bp long including 1 to 29 repeats of the GTGCACCT motif often exhibiting heteroplasmy. The remaining functional proteins within the mitochondria, and those used to replicate it, are encoded in the nuclear genome.
Mitochondria are clonally replicated within the cell and occur in thousands of copies, composing up to 70% of the cytoplasm. The mitochondria are only passed to the offspring through the egg, although there is evidence for an occasional transfer through sperm. As a consequence of its biology, there are several important features of mitochondrial genome that govern its variation: (1) non-Mendelian maternal inheritance, (2) non-recombination, (3) faster evolution rate, and (4) lower effective population size. There are more variants per bp of sequence in the mitochondrial genome compared to nuclear loci because the sequence evolution rate is 5- to 15-fold higher than in the nuclear genome. The mtDNA genome may not always be useful for reconstructing population history because all mitochondrial genes are inherited as one unit. Consequently, even studies that sequence the entire mt genome only reflect the lineage of one locus. Further, the mtDNA may not reflect gene flow in the nuclear genome. Even in the case where two populations are fixed for two different mtDNA haplotypes, if dispersal is primarily through males, there may be no population structure among nuclear loci. Despite these issues, mtDNA loci have provided much insight into horse evolution and history.
Phylogenetics of Equus
The first studies that examined horse mtDNA attempted to resolve the rapid radiation of modern equids. Initially, estimates of divergence were made from restriction enzyme studies, which generated sequence divergence estimates of around 2% per million years, found that the horse was the basal in the Equus group, and clustered the wild asses with zebras. However, these studies failed to resolve the other relationships in this genus. The Przewalski’s haplotype was grouped with the domestic horse, suggesting that it may not be a distinct species (Jansen et al., 2002). Additional studies sequenced a few gene segments, including the variable control region and 12S rRNA, and found further evidence supporting the above patterns (Oakenfull et al., 2000). In 1994, the entire mt genome of horses was sequenced. The gene content was identical to other mammals (Xu & Arnason 1994). The horse mt genome was compared to the donkey (Equus asinus) and exhibited an overall nucleotide difference of 6.9%, with highest divergence in the control region (11.2%), followed by the 13 protein coding genes (8.0%), 2 rRNA genes (4.1%), with the 22 tRNA genes being most conserved (3.5%) (Xu et al., 1996). The greatest amino acid divergence in coding genes was in NADH6 and ATP8 (Xu et al., 1996); both of these are proteins under positive selection across many mammals (da Fonseca et al., 2008).
Over the next decade most of the mtDNA studies used the control region to explore diversity of different breeds (Hill et al., 2002; Jansen et al., 2002). There were high levels of haplotype diversity observed – for example, 17 haplotypes among 100 thoroughbreds sampled (Hill et al., 2002). The findings have led to increased interest in determining whether these patterns reflected multiple domestication events or repeated introgression from the now extinct wild populations. Several studies examined mtDNA diversity in great detail, sequencing the control region in wider array of breeds and individuals (Jansen et al., 2002). In most breeds there were a large number of haplotypes that did not structure geographically.
Several landmark studies compared the mtDNA of archeological and subfossil horse segments with contemporary breeds. These included studies that sequenced ancient samples from Alaska, Europe, and Asia (Jansen et al., 2002; Cieslak et al., 2010; Priskin et al., 2010). In studies with large sample sizes, the mtDNA diversity of both ancient and contemporary horses was high, offering supporting evidence that the maternal lineages present in the domestic horse are the result of both high levels of ancestral variation and frequent maternal introgression from wild populations across the Eurasia (Cieslak et al., 2010). Most of these studies were based on the control region that is highly variable and has high levels of mutation rates that can mask population history (Cieslak et al., 2010; Priskin et al., 2010). The most recent studies reexamined the questions on the domestication of horses, patterns on introgression, and the number of matrilines that were involved in the formation of the domestic horse (Lippold et al., 2011; Achilli et al., 2012). These studies sequenced up to 83 entire mt genomes and supported patterns found previously (Achilli et al., 2012), indicating that there were many diverse mares founding the domestic populations, large amounts of dispersal across Eurasia, coalescence of modern haplotypes to around ∼90,000–160,000 years before present (ybp), and a strong signal for population expansion around 5,000–8,000 ybp consistent with cultural evidence for domestication (Lippold et al., 2011; Achilli et al., 2012). The high level of mt genome diversity in the horse exceeds that of any other domestic animal.
New research directions
To date, the research on mt genome of horses has been limited to using these loci as markers for tracking the evolution, domestication, and population structure of horses. The future research directions on equine mtDNA are in the biology of the mitochondrion and the functional aspects of mt genomics. The mt proteins are important in the physiology of horses as they directly affect ATP production, and therefore many of these should be under selection. This suggests a mitochondrial genomic contribution to athletic performance. In addition, mt mutations have been found to cause a diverse array of disease in humans, including diplopia, ataxia, and Pearson syndrome. Mt disorder can have neurological, cardiac, respiratory, gastrointestinal, endocrinal, and ophthalmological manifestations. Considering the importance of mt genome for cellular respiration, the positive selection detected in genes such as NADH4, NADH5, and NADH6 across mammals, and the diverse array of diseases in humans caused by mt mutations, equine mt genomics is a fertile field yet to be fully explored.
Acknowledgments
Support provided by the Texas A&M CVM Link Equine Research Endowment and USDA grant # 2007-35604-17946 is gratefully acknowledged.
References
Achilli, A., Olivieri, A., Soares, P., Lancioni, H., Hooshiar Kashani, B. et al. (2012). Mitochondrial genomes from modern horses reveal the major haplogroups that underwent domestication. Proceedings of the National Academy of Sciences of the United States of America, 109, 2449–2454.
Bloom, S. E., & Goodpasture, C. (1976). An improved technique for selective silver staining of nucleolar organizer regions in human chromosomes. Human Genetics, 34, 199–206.
Bowling, A. T., & Millon, L. V. (1990). Two autosomal trisomies in the horse: 64,XX,-26,+t(26q26q) and 65,XX,+30. Genome, 33, 679–682.
Caspersson, T., Zech, L., Johansson, C., & Modest, E. J. (1970). Identification of human chromosomes by DNA-binding fluorescent agents. Chromosoma, 30, 215–227.
Chowdhary, B. P., & Raudsepp, T. (2000). Cytogenetics and physical gene maps. In A. T. Bowling & A. Ruvinsky (eds.), The Genetics of the Horse (pp. 171–242). Wallingford, Oxon: CABI.
Cieslak, M., Pruvost, M., Benecke, N., Hofreiter, M., Morales, A. et al. (2010). Origin and history of mitochondrial DNA lineages in domestic horses. PLoS One, 5, e15311.
da Fonseca, R. R., Johnson, W. E., O’Brien, S. J., Ramos, M. J., & Antunes, A. (2008). The adaptive evolution of the mammalian mitochondrial genome. BMC Genomics, 9, 119.
de la Seña, C., Chowdhary, B. P., & Gustavsson, I. (1995). Localization of the telomeric (TTAGGG)n sequences in chromosomes of some domestic animals by fluorescence in situ hybridization. Hereditas, 123, 269–274.
Dionne, F. T., Turcotte, L., Thibault, M. C., Boulay, M. R., Skinner, J. S. et al. (1993). Mitochondrial DNA sequence polymorphism, VO2max, and response to endurance training. Medical Science of Sports Exercise, 25, 766–774.
Gluhovschi, N., Bistriceanu, M., & Palicica, R. (1975). Les troubles de la reproduction chez les animaux domestique dus à des modifications du genome. Medecine Veterinaire, 44, 155–163.
Gluhovschi, N., Bistriceanu, M., Suciu, A., & Bratu, M. (1970). A case of intersexuality in the horse with type 2A+XXXY chromosome formula. The British Veterinary Journal, 126, 522–525.
Harrison, S. P., & Turrion-Gomez, J. L. (2006). Mitochondrial DNA: an important female contribution to thoroughbred racehorse performance. Mitochondrion, 6, 53–63.
Hill, E. W., Bradley, D. G., Al-Barody, M., Ertugrul, O., Splan, R.K. et al. (2002). History and integrity of thoroughbred dam lines revealed in equine mtDNA variation. Animal Genetics, 33, 287–294.
International System for Cytogenetic Nomenclature of the domestic Horse (ISCNH), Bowling, A. T., Breen, M., Chowdhary, B. P., Hirota, K. et al. (1997). Report of the Third International Committee for the Standardization of the domestic horse karyotype, Davis, CA, 1996. Chromosome Research, 5, 433–443.
Jansen, T., Forster, P., Levine, M. A., Oelke, H., Hurles, M. et al. (2002). Mitochondrial DNA and the origins of the domestic horse. Proceedings of the National Academy of Sciences of the United States of America, 99, 10905–10910.
Jiang, F., Miao, Y.-W., Liu, B., Shen, X., Ren, J.-F. et al. (2008). Mitochondrial introgressions into the nuclear genome of the domestic horse. Zoological Research, 29, 577–584.
Khrapko, K. (2011). The timing of mitochondrial DNA mutations in aging. Nature Genetics, 43, 726–727.
Kubien, E. M., Pozor, M. A., & Tischner, M. (1993). Clinical, cytogenetic and endocrine evaluation of a horse with a 65,XXY karyotype. Equine Veterinary Journal, 25, 333–335.
Kumar, D. P., & Sangeetha, N. (2009). Mitochondrial DNA mutations and male infertility. Indian Journal of Human Genetics, 15, 93–97.
Lippold, S., Matzke, N. J., Reissmann, M., & Hofreiter, M. (2011). Whole mitochondrial genome sequencing of domestic horses reveals incorporation of extensive wild horse diversity during domestication. BMC Evolutionary Biology, 11, 328.
Long, S. E. (1996). Tandem 1;30 translocation: a new structural abnormality in the horse (Equus caballus). Cytogenetics and Cell Genetics, 72, 162–163.
Makino, S. (1942). The chromosomes of the horse (Equus caballus). Cytologia, 13, 26–38.
Makino, S., Sofuni, T., & Sasaki, M. S. (1963). A revised study of the chromosomes of the horse, the ass and the mule. Proceedings of the Japanese Academy, 39, 176–181.
Marki, U., & Osterhoff, D. R. (1983). Horse lymphocyte cell synchronization: Improved technique for chromosome analysis. Journal of South African Veterinary Association, 54, 223–224.
Messier, P. E., Drouin, R., & Richer, C. L. (1989). Electron microscopy of gold-labeled human and equine chromosomes. Journal of Histochemistry and Cytochemistry, 37, 1443–1447.
Molteni, L., Cribiu, E. P., De-Giovanni-Machi, A., Beltrami, E., & Succi, G. (1982). The R-banded karyotype of the domestic horse. Paper presented at the Proceedings of the 5th European Colloquium on Cytogenetics of Domestic Animals (Milan).
Moorhead, P. S., Nowell, P. C., Mellman, W. J., Battips, D. M., & Hungerford, D. A. (1960). Chromosome preparations of leukocytes cultured from human peripheral blood. Experimental Cell Research, 20, 613–616.
Oakenfull, E. A., Lim, H. N., & Ryder, O. A. (2000). A survey of equid mitochondrial DNA: Implications for the evolution, genetic diversity and conservation of Equus. Conservation Genetics, 1, 341–355.
Power, M. (1987). The chromosomes of the horse:Karyotype definition, clinical applications and comparative interspecies studies. PhD Thesis, National University of Ireland, Dublin.
Power, M. M. (1990). Chromosomes of the horse. In R. A. McFeely (ed.), Advances in veterinary science and comparative medicine, domestic animal cytogenetics (pp. 131–167). San Diego, CA: Academic Press.
Power, M. M. (1991). The first description of a balanced reciprocal translocation [t(1q;3q)] and its clinical effects in a mare. Equine Veterinary Journal, 23, 146–149.
Priskin, K., Szabo, K., Tomory, G., Bogacsi-Szabo, E., Csanyi, B. et al. (2010). Mitochondrial sequence variation in ancient horses from the Carpathian Basin and possible modern relatives. Genetica, 138, 211–218.
Ricchetti, M., Tekaia, F., & Dujon, B. (2004). Continued colonization of the human genome by mitochondrial DNA. PLoS Biology, 2, E273.
Richer, C. L., Drouin, R., & Messier, P. E. (1989). Banding of horse chromosomes for electron microscopy. Paper presented at the 6th North American Colloquium on Cytogenetics of Domestic Animals, Purdue.
Romagnano, A., Richer, C. L., & Betteridge, K. J. (1983). R-banded chromosomes of the domestic horse obtained after bromodeoxyuridine incorporation by the fluorescence-photolysis-Giemsa technique. Journal of Dairy Science, 66(Suppl. 1), 251.
Rønne, M., Gyldenholm, A. O., & Storm, C. O. (1993). The RBG-banded karyotype of Equus caballus at the 525-band stage. Hereditas, 118, 195–199.
Rothfels, K. H., Alexrad, A. A., Siminovitch, L., & McCulloch Parker, R. C. (1959). The origin of altered cell lines from mouse, monkey and man, as indicated by chromosome and transplantation studies. Proceeding of Canadian Cancer Research Conference, 3, 189–214.
Saxena, R., de Bakker, P. I., Singer, K., Mootha, V., Burtt, N. et al. (2006). Comprehensive association testing of common mitochondrial DNA variation in metabolic disease. American Journal of Human Genetics, 79, 54–61.
Seabright, M. (1971). A rapid banding technique for human chromosomes. Lancet, 2, 971–972.
Taylor, R. W., & Turnbull, D. M. (2005). Mitochondrial DNA mutations in human disease. National Review of Genetics, 6, 389–402.
Trujillo, J. M., Stenius, C., Christian, L. C., & Ohno, S. (1962). Chromosomes of the horse, the donkey, and the mule. Chromosoma, 13, 243–248.
Turner, C., Killoran, C., Thomas, N. S., Rosenberg, M., Chuzhanova, N. A. et al. (2003). Human genetic disease caused by de novo mitochondrial-nuclear DNA transfer. Human Genetics, 112, 303–309.
Tyynismaa, H., & Suomalainen, A. (2009). Mouse models of mitochondrial DNA defects and their relevance for human disease. EMBO Report, 10, 137–143.
Wallace, D. C. (2010). Mitochondrial DNA mutations in disease and aging. Environtal Molecular Mutagens, 51, 440–450.
Xu, X., & Arnason, U. (1994). The complete mitochondrial DNA sequence of the horse, Equus caballus: Extensive heteroplasmy of the control region. Gene, 148, 357–362.
Xu, X., Gullberg, A., & Arnason, U. (1996). The complete mitochondrial DNA (mtDNA) of the donkey and mtDNA comparisons among four closely related mammalian species-pairs. Journal of Molecular Evolution, 43, 438–446.
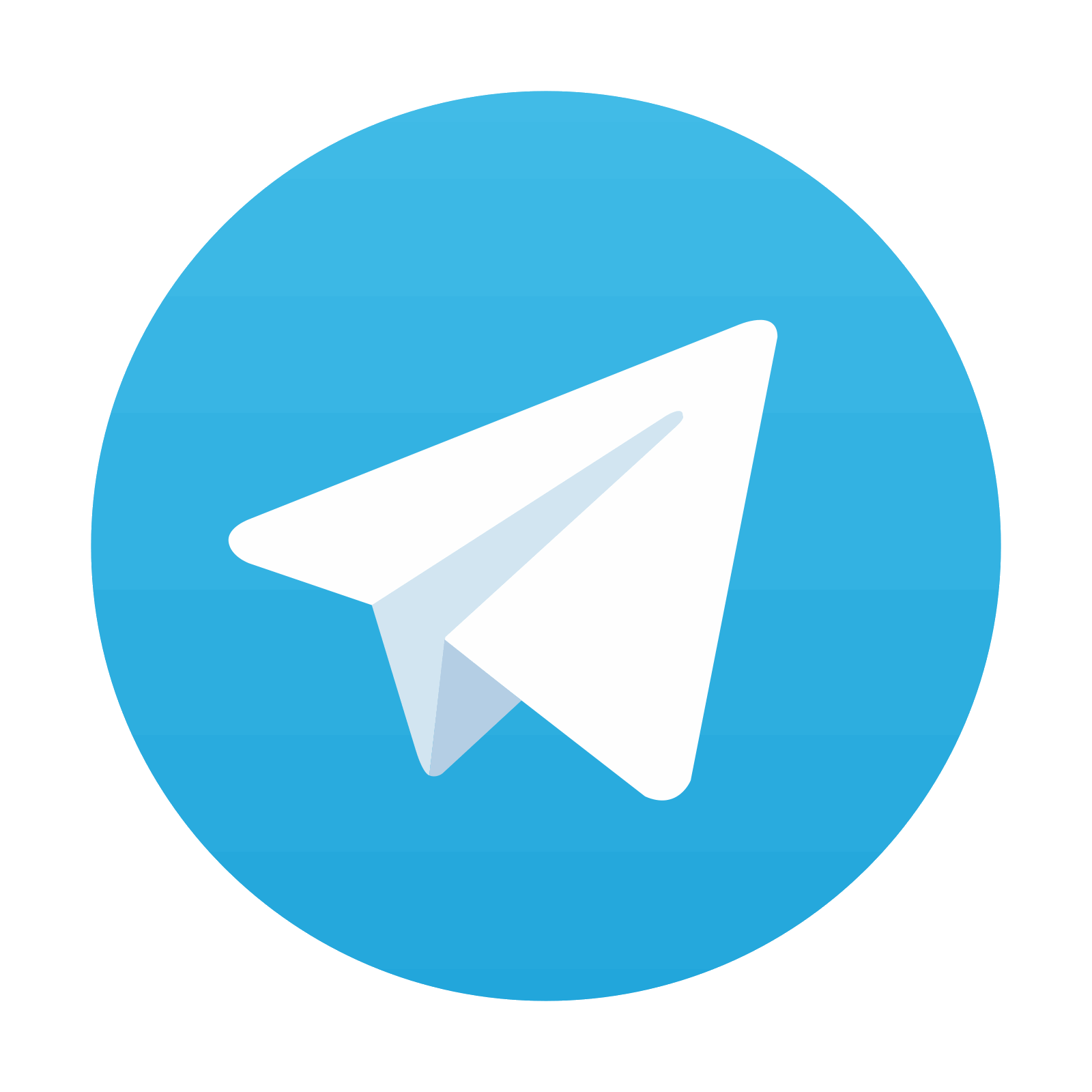
Stay updated, free articles. Join our Telegram channel
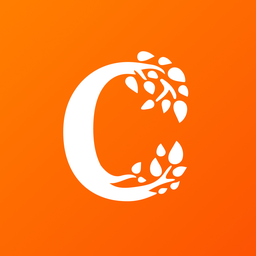
Full access? Get Clinical Tree
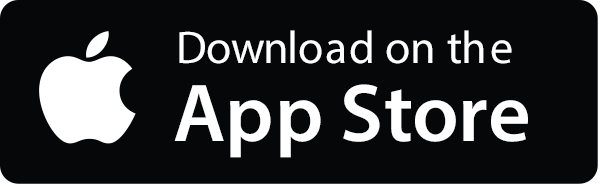
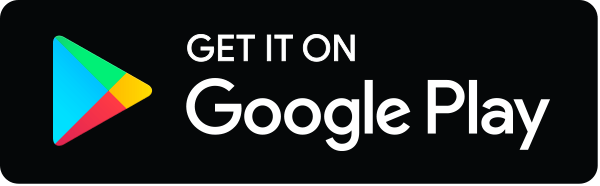