50 Katrina L. Mealey A patient’s response to a drug includes both beneficial (therapeutic) effects as well as any adverse effects, including lack of therapeutic efficacy. While the goal of drug therapy is to produce a specific pharmacological effect without producing adverse effects, it is often difficult to predict how effective or how safe a medication may be for a particular patient. If 10 patients with the same disease were administered the same drug, each might respond differently with respect to both drug efficacy and the likelihood of an adverse reaction. A number of factors may influence a patient’s response to drug therapy, including the patient’s age, species, concurrent medications, diet, health or disease status, and others. Many of these are discussed in Chapter 2 of this text. However, consideration of all of these factors is often not sufficient to explain the degree of interpatient variation observed. The observed interpatient variability in drug response may result from genetically determined differences in drug metabolism, drug distribution, and/or receptors or drug target proteins. The branch of pharmacology that involves identifying genetic variations leading to interindividual differences in drug response is called pharmacogenetics. Pharmacogenetics is likely the ultimate way to establish the right drug and dose for each patient, thereby optimizing efficacy and minimizing toxicity. Despite the fact that this branch of pharmacology is still in its infancy as a science, a number of important discoveries have already contributed to improved pharmacotherapy in human and veterinary patients. The term pharmacogenetics is often used interchangeably with the term pharmacogenomics. Strictly speaking, pharmacogenetics refers to monogenetic (single gene) variants that affect a patient’s response to a particular drug. An example of a pharmacogenetic study might be one that examines the influence of mutations in the thiopurine methyl transferase gene on the response to azathioprine. Pharmacogenomics, on the other hand, refers to the entire spectrum of genes that are involved in determining a patient’s response to a particular drug. An example of a pharmacogenomic study would be one that examines the interaction between cytochrome P450 genes, β1, β2, α1, and α2-adrenergic receptor genes, and drug transporter (i.e., MDR1) genes on the response to carvedilol. The relatively few reports of gene–drug responses in veterinary medicine are pharmacogenetic in nature. While dramatic alterations in drug response have been identified in pharmacogenetic studies, a patient’s overall response to a particular drug will likely require a pharmacogenomics approach to characterize (and summate) the individual contribution of multiple genes to drug response. For the purposes of this chapter, the terms pharmacogenetics and pharmacogenomics will be used interchangeably. The concept that inheritance might explain individual variation in susceptibility to adverse drug reactions and/or variation in drug efficacy was proposed in 1957. The term pharmacogenetics was coined shortly afterward, in 1959. Relatively little pharmacogenetic research occurred for the next three decades. A resurgence in pharmacogenetic research occurred coincidentally with the initiation of the Human Genome Project in 1990. Since then, research in pharmacogenetics has expanded at a remarkable rate. There are now at least five journals exclusively devoted to pharmacogenetic/pharmacogenomic research, and many other medical and pharmacological journals encouraging submissions reporting results of pharmacogenetic research. Following completion of the Human Genome Project (announced in 2003), the National Human Genome Research Institute challenged investigators to develop genome-based approaches to predict drug response, setting the stage for continued growth in the field of pharmacogenetics. The human genome contains approximately 3 billion nucleotide bases, representing roughly 30,000 genes. When a gene is expressed, DNA is transcribed into RNA, which is then translated to make proteins. Three consecutive nucleotide bases form a specific codon, specifying the addition of a particular amino acid or signaling amino acid chain termination (stop codons). The genetic code is said to have redundancy, which simply means that there may be two or more different codons for the same amino acid. In humans, for example, both GGA and GGC code for the amino acid glycine. Redundancy exists in the canine genome as well, with TAA, TGA, and TAG all representing stop codons. A gene is simply the DNA sequence containing a series of codons that specify a particular protein. The variation that occurs between individuals in a population is a result sequence differences in specific genes. These sequence differences are often the result of mutations. A mutation alters the sequence of nucleotide bases in a DNA molecule. This in turn alters the transcribed RNA, creating a different codon. Some mutations, because of the redundancy described above, are silent, meaning that if the mutation results in a base change that creates a codon for the same amino acid (i.e., GGA to GGC) as specified by the original DNA sequence, there is no change in protein structure or function. However, if the mutation results in a different amino acid, or the creation of a stop codon, the change in protein structure and function can be deleterious. At each gene locus, an individual carries two alleles, one from each parent. An allele is defined as the DNA sequence at a given gene’s location on the chromosome. If an individual has two identical alleles, that individual is said to have a homozygous genotype. If an individual has two different alleles, that individual is said to have a heterozygous genotype. The phenotype of each individual with regard to a specific gene is the outward, physical manifestation of a given genotype. That outward physical manifestation might be something immediately obvious in a given individual such as eye color, or it may not be apparent until a particular drug is administered to that individual. Variations in a given gene may be present rarely in a population, or in relatively large numbers in a population. Polymorphisms are defined as genetic variations occurring at a frequency of 1% or greater in the population (species of interest). In humans, many of the genes encoding cytochrome P450 enzymes are polymorphic (specific mutations are present in greater than 1% of the population), whereas some inherited human diseases such as cystic fibrosis are caused by rare mutations occurring in less than 1% of the population. For some diseases, identification of a specific mutation may be used to provide specific treatment approaches for the patient and, in the case of veterinary patients, guide breeding decisions as well. However, many common diseases in human patients, such as diabetes mellitus and many cardiovascular disease, are polygenic (more than one gene contributes to the disease). For diseases that are polygenic in nature, the pathophysiology of the disease is complex, and specific treatments based on particular mutations will be difficult to sort out. It is likely that many important diseases in veterinary medicine (i.e., hip dysplasia, epilepsy, most types of cancer) are polygenic also. Genetic variation can affect both the pharmacokinetics (i.e., drug absorption, distribution, metabolism, and excretion) and pharmacodynamics (i.e., interaction with drug transporters and receptors) of pharmaceutical agents. The concept of pharmacogenetics originated in the 1950s when it was discovered that the antimalarial drug primaquine caused hemolysis in a subpopulation of individuals (Hochstein, 1971). Ultimately, it was shown that the enzyme glucose 6-phosphate dehydrogenase had reduced function in affected individuals as compared to the majority of the population (Hochstein, 1971). At the time, techniques to study molecular biology were not available, so the field of pharmacogenetics was initially based purely on phenotypic observations (measurement of enzyme function). Discovery of the specific mutation in the glucose 6-phosphate dehydrogenase gene would not occur until a few decades later. With the rapid advancement of molecular techniques, modern pharmacogenetic research differs significantly from those initial phenotypic observations. It currently involves identifying both the phenotype and the genetic variation responsible for it. Researchers perform systematic searches to identify functionally significant variations in DNA sequences in genes that affect drug disposition. In many instances, the genetic variation in a gene is identified before the phenotypic consequence is known. The availability of published sequences for the canine genome, feline genome, and genomes of other veterinary species has accelerated the progress of pharmacogenetic discoveries, facilitating the ultimate goal of pharmacogenetics, which is individualization of drug therapy. It is important to note that individualization of drug therapy encompasses two distinct, yet equally important, clinical implications. First is the ability to predict those patients at high risk for developing drug toxicity. These patients may have a mutation in a drug-metabolizing enzyme that decreases drug clearance. For such patients a lower drug dose, longer dosing interval, or alternate drug should be administered. Second is the ability to predict those patients that are most likely to benefit from a particular drug because of appropriate receptor interactions. Patients with mutations in drug receptors may be poor responders to certain pharmaceutical agents. Rather than using a trial-and-error approach to drug therapy, a veterinarian could select the drug most likely to produce the desired pharmacological response in a particular patient, decreasing the amount of time in which the patient’s disease state is poorly controlled. Described in this chapter are several recent discoveries in veterinary pharmacogenetics and examples of pharmacogenetically based differences in drug absorption, distribution, metabolism, excretion, and drug–receptor interactions. The fundamentals of these topics were extensively discussed in Chapters 2, 3 and 4. The impact of these discoveries in clinical veterinary medicine will also be presented. Systemic bioavailability of orally administered drugs is often considered to be a function of physicochemical characteristics of the drug and subsequent hepatic metabolism. A number of other factors have recently been shown to impact the ability of a drug to be absorbed into the systemic circulation after oral administration. Intestinal Phase I drug metabolism and active drug extrusion by efflux transporters are now considered to be among the most important determinants of oral drug bioavailability. Consequently, genetic variation in intestinal drug metabolizing enzymes and drug transporters as well as hepatic drug metabolizing enzymes and transporters is likely to dramatically affect oral drug absorption. In people, CYP3A is expressed at higher levels in mature villus tip enterocytes than in hepatocytes (Patel and Mitra, 2001). Because intestinal villi comprise such a large surface area, there is a high likelihood that absorbed drug will interact with intestinal CYP3A enzyme, facilitating substantial first-pass metabolism. Interpatient variability in intestinal CYP3A levels has been studied in a small sample of human patients. Elevenfold variations in CYP3A protein content and sixfold variation in enzymatic activity were identified, suggesting that CYP3A polymorphisms exist in the human population (Scordo and Spina, 2002). While intestinal drug metabolism is thought to be important in veterinary patients also, relatively little is known with regard to interpatient variability in enzyme activity. Drug transporters are also known to play an important role in drug absorption. Many drug transporters have been identified in people, but the most well-characterized drug transporter is P-glycoprotein (P-gp), the product of the MDR1 (also known as the ABCB1) gene. The potential impact of transporter pharmacogenetics on drug pharmacokinetics is dramatically illustrated by P-gp. P-gp is a transmembrane protein that was first described in highly resistant tumor cell lines (Roninson, 1992). Tumor cells expressing P-gp were cross-resistant to various anticancer agents (anthracyclines, vinca alkaloids, taxanes, and others). P-gp has since been shown to act as an ATP-dependent pump that exports drugs from nonneoplastic cells as well. In normal mammalian tissues, P-gp appears to function in a protective capacity. P-gp is expressed on bile canaliculi, renal tubular epithelial cells, the placenta, brain capillary endothelial cells, and at the luminal border of intestinal epithelial cells (Thiebaut et al., 1987). At these locations, P-gp pumps selected drugs either out of the body (into the bile, urine, or intestinal lumen) or away from protected sites (brain tissue, fetus). The significant role intestinal P-gp can play in determining oral drug bioavailability has been demonstrated in rodent studies. In mdr1 (−/−) knockout mice, oral bioavailability of many P-gp substrate drugs (vinblastine, taxol, digoxin, loperamide, ivermectin, cyclosporine A, and others) is substantially greater than in wild-type mice (Schinkel et al., 1995; Sills et al., 2002). Similarly, MDR1 polymorphisms in people have been shown to result in altered oral bioavailability of P-gp substrate drugs. Studies have shown that oral bioavailability of digoxin, a P-gp substrate, is greater in subjects with the MDR1 3435TT genotype compared with those with the MDR1 3435CC genotype (Verstuyft et al., 2003). The P-gp substrate phenytoin has also been shown to have lower oral bioavailability in subjects with the MDR1 3435CC genotype (Kerb et al., 2001). P-gp has been fairly well characterized in dogs. The tissue distribution of P-gp in dogs is similar to that in people (Ginn, 1996), and it has been shown to contribute to chemotherapeutic drug resistance in vitro and in vivo (Mealey et al., 1998; Page et al., 2000; McEntee et al., 2003). Its role in determining oral drug bioavailability in dogs has been characterized only indirectly until recently. The indirect evidence to support that P-gp is important in oral drug absorption in dogs was generated from studies using P-gp inhibitors. Bioavailability of the anticancer agent (and P-gp substrate) docetaxel was increased 17-fold when coadministered with a P-gp inhibitor (McEntee et al., 2003). More recently, P-gp’s role in oral drug absorption was investigated in dogs affected by an important polymorphism of the MDR1 (ABCB1) gene. This polymorphism consists of a four-base-pair deletion mutation (ABCB1-1Δ). This deletion results in a shift of the reading frame that generates several premature stop codons (Mealey et al., 2001). Because protein synthesis is terminated before even 10% of the protein product is synthesized, dogs with two mutant alleles exhibit a P-gp null phenotype, similar to mdr1 (−/−) knockout mice. Affected dogs include many herding breeds. For example, roughly 75% of collies in the United States, France, and Australia have at least one mutant allele (Mealey et al., 2002). Other affected herding breeds, albeit at a lower frequency, include Old English Sheepdogs, Australian Shepherds, Shelties, English Shepherds, Border Collies, German Shepherds, Silken Windhounds, McNabs, and Long-haired Whippets (Neff et al., 2004). Oral bioavailability of several P-gp substrates (quinidine, loperamide, nelfinavir, and cyclosporine) were determined in MDR1 mutant/mutant and MDR1 normal/normal dogs (Figure 50.1). Contrary to what appears to be the case in other species, there were no differences in oral bioavailability between MDR1 mutant/mutant dogs and MDR1 normal/normal dogs for any of the drugs studied (Mise et al., 2004). There are several explanations for the apparent discordant results. First, many P-gp substrates are also CYP3A substrates. Variable CYP3A activity in the study population could have masked differences in P-gp-mediated drug absorption. Second, there may be species differences in P-gp substrate binding and/or efflux kinetics such that oral drug bioavailability is impacted in one species with deficient intestinal P-gp function but not in another. For example, rodents have two genes that encode for P-gp (abcb1a and abcb1b) while dogs have only one (ABCB1). Lastly, at concentrations of drug achieved in the intestine after oral drug administration, P-gp may simply be saturated. Figure 50.1 Plasma concentration versus time curves of cyclosporine after oral administration (4 mg/kg) to MDR1 mutant/mutant (open symbols, dashed lines; n = 3) and MDR1 normal/normal (closed symbols, solid lines; n = 2) Collie dogs. P-glycoprotein is not the only drug transporter that has been shown to affect oral drug absorption in humans and rodents. The breast cancer resistance protein (BCRP), encoded by the ABCG2 gene, affects oral bioavailability of several drugs in abcg2 knockout mice and/or human patients with functional ABCG2 polymorphisms. Patients with an ABCG2 variant that alters amino acid 141 have greater oral bioavailability of topotecan than patients with the wild-type allele. Similarly, this variant is associated with greater oral bioavailability of rosuvastatin. Domestic cats, compared to 10 other mammalian species, have defective ABCG2 function as a result of an ABCG2 variant that alters amino acid 149 (Court, 2013b). Whether or not this variant affects oral absorption of ABCG2 substrate drugs is not yet known. Drug distribution, the delivery of drugs from the systemic circulation to tissues, can be dramatically affected by pharmacogenetics. The drug transporter P-gp serves as an important barrier to the distribution of substrate drugs to selected tissues. For example, P-gp is a component of the blood–brain barrier, the blood–testes barrier, and the placenta. Therefore, distribution of P-gp substrate drugs to these tissues is greatly enhanced in dogs with the MDR1 deletion mutation. Dogs homozygous for the deletion (MDR1 mutant/mutant) experience adverse neurological effects after a single dose of ivermectin (120 µg/kg). Heterozygous (MDR1 wild-type/mutant) or homozygous wild-type dogs are not sensitive to ivermectin neurotoxicity at the 120 µg/kg dose, but heterozygote animals may experience neurotoxicity at ivermectin doses greater than 300 µg/kg, particularly if daily doses are administered (i.e., protocols for treatment of demodectic mange). Dogs homozygous for the normal MDR1 allele (MDR1 normal/normal), can receive 2000 µg/kg as a single dose without signs of toxicity, and can receive 600 µg/kg/day for months without signs of toxicity. Dogs with the MDR1 mutation also appear to have increased susceptibility to neurological adverse effects of other avermectins, including milbemycin, selamectin (Revolution® package insert), and moxidectin (Tranquilli et al., 1991). Interestingly, a retrospective study conducted by a national veterinary poison center reported that Collies (the breed with the highest frequency of the MDR1 mutation) were overrepresented in canine cases of loperamide-induced neurotoxicity (Hugnet et al., 1996). Many Collies displayed signs of neurological toxicity after administration of routinely recommended doses of the antidiarrheal agent loperamide (Imodium®). Loperamide is an opioid that is generally devoid of CNS activity because it is excluded from the brain by P-gp (Ericsson and Johnson, 1990; Wandel et al., 2002). One study demonstrated that dogs with the MDR1 mutation experienced marked CNS depression after loperamide administration whereas MDR1 normal/normal dogs experienced no CNS depression (Court et al., 1999). Figure 50.2 dramatically illustrates P-gp’s ability to limit substrate accumulation within the CNS. MDR1 mutant/mutant and MDR1 normal/normal dogs received a single intravenous dose of 99mTc-sestamibi, a radiolabeled P-gp substrate. Nuclear scintigraphy demonstrated minimal uptake of radioactivity in the brain of MDR1 normal/normal dogs because P-gp actively effluxes the substrate. Sensitive brain tissue is therefore protected from potentially toxic compounds. Conversely, uptake of radioactivity in the brain of MDR1 mutant/mutant dogs is indistinguishable from surrounding tissues, illustrating the importance of P-gp as a component of the blood–brain barrier (Court et al., 1999). Brain tissue is exposed to much higher concentrations of potentially toxic compounds. Figure 50.2 Nuclear scintigraphy was used to image the head of two Collies after intravenous injection of the P-gp substrate 99mTc-sestamibi. The MDR1 normal/normal dog (A) with functional P-gp has prevented accumulation of labeled substrate in the brain, while the MDR1 mutant/mutant dog (B) with defective P-gp function has accumulated the P-gp substrate within brain tissue (Mealey et al., 2008). Less information is available regarding P-gp and the blood brain–barrier in cats. The author has recently investigated the feline ABCB1
Pharmacogenomics
Basic Genetic Concepts
Pharmacogenetics
Pharmacogenetics of Oral Drug Absorption
Pharmacogenetics of Drug Distribution
Stay updated, free articles. Join our Telegram channel
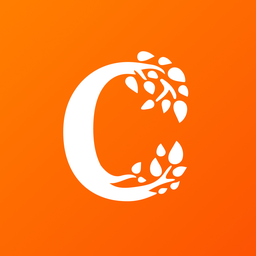
Full access? Get Clinical Tree
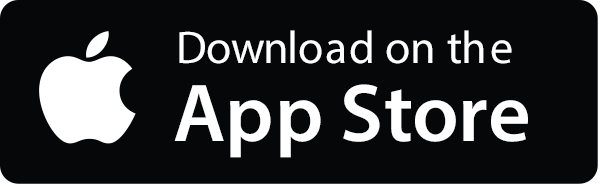
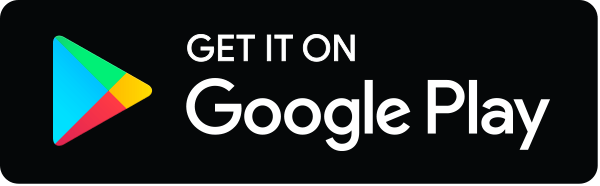