13 Butch KuKanich and Mark G. Papich The opioids include drugs that are true opiates – derivatives of opium – and drugs that are synthetic agents but also affect opiate receptors. Opiates include morphine, codeine, and thebaine isolated from the poppy plant. These drugs differ among one another primarily in potency, but also may have different pharmacokinetic and pharmacodynamic properties. Opioids include all the opiates, as well as synthetic drugs, such as fentanyl, methadone, and meperidine (pethidine) that are not chemically related to morphine but act as opiate receptor agonists. Various types of opiate receptors will be discussed later in the chapter. The opioids are valuable tools in the veterinarian’s armamentarium of drugs. Although most of the drugs in this group are controlled substances because of their potential for abuse by humans, they can have profound effects for treatment of animals (Table 13.1 ). There are many overlapping areas with other therapeutic uses discussed in other chapters of this book (for example Chapter 46 dealing with gastrointestinal drugs and Chapter 48 dealing with respiratory drugs – antitussives). Table 13.1 Therapeutic uses of opioids in animals An important advantage of opioid analgesic drugs is their high efficacy and remarkable safety. If adverse effects are recognized, their short half-lives in animals usually produce a rapid alleviation of clinical signs. If adverse reactions are severe (e.g., dysphoria or life-threatening respiratory depression) these drugs also have the benefit of reversibility, which is accomplished quickly with administration of an opioid antagonist such as naloxone. This lack of serious adverse effects allows clinicians to gradually escalate doses of opioid agonists in patients as needed for pain or decrease dose if adverse effects occur in highly sensitive animals. Opioid agonists/antagonists may have a ceiling effect limiting the effectiveness of high doses, as discussed later. A nociceptive stimulus produces an unpleasant sensation. Pain is the coupling of the unpleasant sensation with conscious perception and eliciting an emotional response. There is debate on whether all animals have true emotional responses, and thus whether they can feel pain according to its definition, but there is no debate that nociception is present in animals. Nociceptive receptors are free nerve endings distributed throughout the body, which detect a nociceptive stimulus. Nociceptive stimuli are transmitted from peripheral nerves though the spinal cord to numerous areas within the central nervous system. Mechanical (pressure, fractures, stretching), temperature (hear or cold), and chemical (including endogenous substances such as inflammatory cytokines and changes in tissue pH) stimuli are all types of stimuli that produce pain. For the discussions in this chapter pain and nociception will be used synonymously. Large strides have been made recently in the recognition and treatment of pain in animals. Numerous pharmacokinetic and pharmacodynamic studies have been performed in order to better gain our understanding of opioids in animals through laboratory and clinical studies. Treatment regimens used in humans cannot be easily extrapolated to animal species treated by veterinarians because of species differences in pain sensation, pharmacokinetic differences, efficacy, and adverse effects of opioids. Opioids are considered the prototypical analgesic, antidiarrheal, and antitussive drugs by which other drugs are compared. Opium was first imported into the United States in 1840, primarily from the country of Turkey. It was widely used to treat a variety of maladies, including pain, diarrhea, and coughing. In the 1870s, because of widespread use after the Civil War, opiate use and addiction was a recognized problem. There are over 20 opiate alkaloids in opium and in the early 19th century, the chemist Sertürner isolated morphine, which he named after Morpheus, the Greek god of sleep and dreams. Heroin was also developed in the early 1900s by the same chemists at Bayer who developed aspirin. It was intended to be a safe and nonaddictive analgesic. There have been other attempts – none completely successful – during the next century to develop drugs as effective as the opiates, but without the abuse and addiction potential. The majority of opioids are now controlled by regulatory authorities. In the United States, the Drug Enforcement Agency (DEA) and Food and Drug Administration (FDA) are the regulatory agencies. (Regulation of drugs is discussed in more detail in Chapter 55. Prescribing controlled drugs is discussed in Chapter 56.) The FDA approves drugs based on efficacy and safety, and drugs with the potential for abuse are scheduled by the DEA on the basis of its addictive potential (Schedule I through V). Schedule I (CI) drugs have no realistic medical benefit (e.g., heroin). Schedule II (CII) drugs can be highly addictive, but have important therapeutic uses. Morphine and most of the pure agonists are listed in Schedule II (Table 13.2 ). Schedule III (CIII) have an abuse potential, but less than CII drugs. Schedule IV (CIV) drugs have an abuse potential, but less than CIII drugs and Schedule V (CV) drugs have an abuse potential, but less than CIV drugs. Occasionally, a drug’s schedule may change. For example, the DEA recently changed hydrocodone combination drugs (e.g., hydrocodone with acetaminophen) from CIII to CII drugs, and tramadol went from being an unscheduled drug to Schedule IV. Table 13.2 United States drug scheduling of the opioid narcotics; complete list may be found at: http://www.usdoj.gov/dea/pubs/scheduling.html. Source: United States Drug Enforcement Administration. In veterinary medicine, the earliest recorded use of opium was in 1815, when it was considered important in the Materia Medica (Stalheim, 1990) (See Chapter 1 for a discussion of the Materia Medica.) In 1930, morphine was first recommended in a veterinary pharmacology textbook for treatment of horses for colic, cough, and analgesia. Although recommended for treatment of pain and colic in horses at that time, the adverse effects in horses were not recognized. Other drugs used in the early 20th century by veterinarians included combinations of morphine and atropine for pain in dogs and the use of paregoric (tincture of opium) for treatment of flatulence. Early veterinary preparations included paregoric, laudanum (opium), ipecac and opium (Diver’s powder), morphine, heroin (0.5 to 2 grains for a horse), codeine, and dionin (ethylmorphine) (Stalheim, 1990). Veterinary medicine has advanced considerably since those early years and it is common for modern veterinary hospitals to include morphine and other potent derivatives in their pharmacy. Injectable solutions for intermittent administration or constant rate infusion, oral formulations, and transdermal delivery devices are common in most veterinary hospitals used as preanesthetics, anesthetic adjuncts, and to provide analgesia among other uses. Three main classes of opiate receptors have been identified: μ, κ, and δ (Table 13.3 ). Numerous opiate receptor subtypes have also been identified. In some textbooks, a sigma (σ) receptor is listed, but the significance of this receptor is not understood and is not considered a true opiate receptor by many pharmacologists. Table 13.3 Opiate receptor types and their associated effects (some textbooks also list the sigma-receptor, but this may not have any significance) ADH, antidiuretic hormone. Clinically, differences in mu (μ)-opioid effects have been observed in dogs with marked pharmacodynamic variability of effects. A morphine dose that produces desirable and predictable effects in most dogs may produce profound sedation in some individuals and marked dysphoria in others. In the past, these ranges of responses have been suggested to be “sensitivity” differences as the doses and plasma concentrations were within expected ranges. However, recent data suggest opioid receptor subtypes exist, which may contribute to the variability in opioid effects, but are not well characterized in dogs. Methadone combined with morphine and codeine produces a synergistic analgesic effect in mice, but only additive effects with other opioids such as oxymorphone and fentanyl, suggesting different receptor binding for morphine and methadone (Bolan et al., 2002). μ-opioid deficient mice (CXBX strain) are insensitive to morphine, but still respond to some other opioids including methadone and fentanyl, which also suggests μ-opioid subtypes exist (Vaught et al., 1988; Chang et al., 1998). Although only one μ-opioid receptor gene has been identified, multiple μ-opioid splice variants have been documented in rats, which may explain differential agonist effects (Verzillo et al., 2014). There have also been single nucleotide polymorphisms identified in the μ-opioid gene of dogs, which may play a role in opioid-induced dysphoria (Hawley and Wetmore, 2010). Endogenous opioids are natural chemicals in the body (peptides) that interact with opiate receptors to produce natural physiological responses, including modulation of pain. β-endorphin is the endogenous opioid peptide with the highest affinity for μ receptors and is derived from proopiomelanocortin. Leucine- and methinone-enkephalin are derived from proenkephalin and are the endogenous ligands for the δ receptor. Dynorphin A is the endogenous ligand for the κ receptor and is derived from prodynorphin. The role of dynorphins remains controversial because they induce sensitization of nociceptive transmission through activation of NMDA receptors. An endogenous opioid peptide, termed nociceptin or orphanin FQ (N/OFQ), with similar homology to dynorphin, has also been described (Meunier et al., 1995; Reinscheid et al., 1995). Orphanin precursors have been identified in the hippocampus, cortex, and numerous sensory sites (Neal et al., 1999). Exogenous N/OFQ administration results in a variety of responses ranging from analgesia to antianalgesia and opioid antagonism. However, N/OFQ antagonists have consistent analgesic properties when administered supraspinally, which may prove to be a useful target for analgesics in the future (Heinricher, 2005). However, no drugs affecting N/OFQ receptors are currently available for use, therefore these drug classes will not be addressed in this chapter. Opioids have been characterized by the type of interaction with the opiate receptor type(s) with which they interact (μ, κ, and δ), and by the effect elicited upon binding (Figure 13.1). Opioids may be full agonists, partial agonists, antagonists, and combinations thereof. An agonist produces a dose-dependent effect which eventually plateaus with unconsciousness. A partial agonist binds to the opiate receptor, but plateaus at a submaximum response (less than a full agonist) despite increasing doses. The opioid antagonists are competitive antagonists which bind to the receptor but do not result in receptor activation and may displace the agonist from the opiate receptor in some cases, resulting in “reversal” of effects. Morphine is a full agonist at the μ receptor whereas buprenorphine is a partial agonist. Nalbuphine is an antagonist at the μ receptor but an agonist κ at the receptor. Naloxone is primarily an antagonist at the μ receptor but also has antagonist effects at the κ and δ receptors. Figure 13.1 Panel A demonstrates two drugs with equal efficacy, but different potency such as fentanyl and morphine. Panel B demonstrates drugs with equal potency, but different efficacy such as fentanyl and buprenorphine. Opioids exert their primary analgesic effect by binding to spinal and supraspinal receptors (Tables 13.3 and 13.4). However, peripheral effects of opioids have been documented by local injection of opioids in joints (intraarticular injection) at doses that do not produce systemic effects and the effect is abolished when injected with naloxone (Stein et al., 1991). Table 13.4 The primary sites of action for opioid and associated drugs used in veterinary medicine 5-HT, serotonin; NMDA, N-methyl-D-aspartate; M1, muscarinic M1; M3, muscarinic M3. ++ agonist; + submaximal agonist; – antagonist; – submaximal antagonist; * via metabolism to morphine; ** via metabolism to O-desmethyltramadol. When an opioid binds to spinal receptors it produces activation of G-coupled proteins (which inhibit adenylyl cyclase), activation of receptor linked K+ ion channels, and inhibition of voltage gated Ca2+ channels. Presynaptic μ, κ, and δ spinal receptors are present in the dorsal horn of the spinal cord, decreasing excitatory neurotransmitter release by decreasing the rate of calcium influx. Postsynaptic μ receptors are also present in the dorsal root ganglion, which hyperpolarize the neuron by increasing potassium channel conductance resulting in a decreased propagation of the nociceptive signal. The supraspinal opioid pathways are poorly understood, but concurrent activation with spinal receptors result in analgesic synergism (Roerig and Fujimoto, 1989; Yeung and Rudy, 1980). Synergism also exists between supraspinal μ and δ receptors when concurrently activated. Microinjection of morphine into the periqueductal gray (PAG) region results in inhibition of γ-amino butyric acid (GABA) interneurons, resulting in activation medullary pain inhibitory pathways (descending pathways) that inhibit dorsal horn nociceptors through release of serotonin (Yaksh and Tyce, 1979). Subsequent studies identified norepinephrine contributing a greater inhibitory effect than serotonin using knockout mice (Hall et al., 2011). Full μ-opioids can provide a more profound analgesic effect than partial μ-agonists or κ-agonists for acute pain. However, partial μ-agonists and κ-agonist can still be effectively used for mild to moderate pain conditions if appropriate doses and dose intervals are chosen. The partial agonists or agonists/antagonists may be preferred in animals that exhibit excitement or dysphoria caused by a pure μ-agonist. Analgesia from systemic opioids are primarily limited to effects on c fiber nociceptors (slow conduction, unmyelinated nociceptors that transmit poorly localized, dull aching pain) when administered at clinical dosages. The Aδ nociceptors (fast conduction, myelinated nociceptors that transmit sharp localized pain) are minimally affected by systemic doses of opioids, which is the reason systemic opioids alone are inappropriate for invasive surgical procedures and anesthesia is required. Epidural opioids may produce high concentrations at the spinal level, which may inhibit both c-fiber and Aδ fiber nociceptors, producing greater analgesia but alone are insufficient for invasive surgical procedures. In contrast, epidural local anesthetics will completely block spinal nerve (and pain) transmission caudal to the injection site and some surgical procedures could be humanely performed. The evaluation of analgesic response to opioids in animals is limited by other properties of the drugs (e.g., sedation) or misinterpretation of response because of dysphoria, panting, or ataxia. Innate behaviors of animals also often confound our ability to interpret responses to opioids. Clinical assessments include subjective measures that may not be indicative of pain. Placebo rates for postoperative pain studies in dogs undergoing soft tissue and orthopedic surgery have been as high as 100%. The extrapolation of experimental models of antinociceptive responses to clinical effects of these drugs is not straightforward and may overestimate or underestimate the degree and duration of analgesia. Nevertheless, many of the dosing regimens discussed in this chapter are made using information obtained from experimental models and clinical trials in the species of interest in addition to extrapolation from other species including humans. Opioids produce a dose-dependent respiratory depression mediated via activation of the μ receptor. Respiratory depression is produced by a decreased response to increases in carbon dioxide partial pressures (PCO2). The respiratory depressant effects of opioids are well tolerated in healthy animals even when supratherapeutic doses are administered. Concurrent anesthesia or other drugs that cause respiratory depression can increase the respiratory depressant effects of opioids (Hug et al., 1981). Respiratory depressant effects may be more problematic in animals with preexisting respiratory disease (asthma, bronchitis, cor pulmonale) or increased intracranial pressure. Neonatal animals (young, healthy, and pain free) tend to be more sensitive to the respiratory depressant effects of opioids, but opioids can still be safely administered to neonates. Opioids cross the placenta and respiratory depressant and sedative effects on the fetus can occur and should be monitored and treated appropriately. Naloxone administered sublingual (or parenterally) to newborns will reverse deleterious opioid effects. High doses of morphine and other opioids produce excitement. Very high doses can produce convulsions. Similarly, rapid IV administration of opioids can cause CNS excitation. In dogs, morphine, 180–200 mg/kg IV, produced grand mal seizures, and convulsive changes in electroencephalogram (EEG) tracings and excitement were seen in dogs administered morphine 20 mg/kg SC (Wikler and Altschul, 1950; de Castro et al., 1979). (Note that therapeutic doses rarely exceed 0.5–1 mg/kg.) Cats respond similarly to high doses of morphine where 20 mg/kg SC produced EEG tracings consistent with convulsive activity and generalized excitement (Tuttle and Elliot, 1969). In veterinary medicine, there are great species differences with respect to excitement caused by opioids. Dogs typically become sedate, whereas cats, horses, and other large animal species are prone to excitement. The explanation for increased susceptibility to these effects in some animals is probably related to the distribution of opiate receptors in certain regions of the brain independent of the drug’s pharmacokinetics. The distribution of opiate receptors in brains of animals that are sedated from opioids (e.g., dogs) is greater than the animals that are more prone to excitement (horses). Alternatively, the excitement may be due to a release of excitatory neurotransmitters or decreased release of inhibitory neurotransmitters. Some anesthesiologists suggest that the reaction may be dopaminergic, adrenergic, or caused by decreased activity of the inhibitory neurotransmitters GABA or glycine (Werz and Macdonald, 1982). Release of acetylcholine also has been suggested as a cause of excitation (Mullin et al., 1973). In another study, it was suggested that excitement in animals may be caused by histamine release, but the doses of morphine exceeded clinically recommended doses in those studies. Morphine administration to dogs induced greater histamine release, and also more excitement compared to oxymorphone after administration of an equianalgesic dose, but the doses of morphine (2 mg/kg IV) were four times higher than recommended clinically (Robinson et al., 1988). When morphine was compared to hydromorphone in dogs (Guedes et al., 2007a), morphine caused a transient increase in plasma histamine and 1/5 dogs also exhibited neuroexcitatory behavior at 0.5 mg/kg IV. By contrast, hydromorphone did not cause increases in histamine but two dogs became excited after 0.1 mg/kg IV. These studies indicate histamine alone is not a cause of opioid-induced CNS excitation. Cats are one of the species that is more sensitive to morphine-induced CNS excitation and dysphoria. There are isolated studies in which cats have tolerated morphine doses up to 2 mg/kg (Barr et al., 2000), but lower clinical doses are generally administered to cats compared to dogs. The differences between dogs and cats are not attributed to pharmacokinetics. Although cats have somewhat lower clearance for some opiates (Table 13.5 ), this difference is not enough to account for opioid-induced excitement in cats. Table 13.5 Pharmacokinetic parameter of opioids administered IV and PO bioavailability in dogs and cats *Oral dose data, no IV dose administered; ** only IM data available, clearance is per bioavailability, volume of distribution is per bioavailability; <LOQ, no measurable plasma concentrations; LD50, lethal dose 50%. Horses are also more sensitive to the excitement caused by this group of drugs. Because of this, pure opioid agonists such as fentanyl and morphine are not recommended to be used alone. Dose-dependent excitation and increased locomotor activity has been observed in horses and may occur before maximum analgesia is obtained with the degree of excitation dependent on the specific opioid, individual variability, and degree (or lack thereof) pain in the horses (Muir et al., 1978; Tobin et al., 1979; Mama et al., 1992). For example buprenorphine, normally well tolerated in dogs and cats, produced restlessness, excitement, head shaking, pawing, and leg shifting at a dose of 5 and 10 μg/kg IV in healthy pain-free animals that was dose dependent (Carregaro et al., 2006, 2007; Messenger et al., 2011; Davis et al., 2012). However, administration of 10 μg/kg IV in separate studies did not produce excitement, but increased locomotor activity was still observed in healthy pain-free horses (Love et al., 2015). Buprenorphine, 10 μg/kg administered as part of a preanesthetic protocol for castration, was well tolerated with no excitement, but increased locomotor activity occurred (Love et al., 2013). Morphine was investigated for its use as an anesthetic adjunct for healthy pain-free horses at doses of 0.25 and 2 mg/kg IV with isoflurane anesthesia (Steffey et al., 2003). The authors concluded that undesirable and dangerous behavior during recovery from 2 mg/kg IV morphine still persisted after 4 hours, and did not support the use of high doses of morphine as an anesthetic adjunct in this regimen. However, the recovery and effects of 0.25 mg/kg morphine were similar to the saline-treated group. When opioids have been used in painful horses, fewer adverse reactions are observed (Mircica et al., 2003; Devine et al., 2013). Only 1 of 75 clinically treated horses administered morphine (0.1 mg/kg IM) had excitation reported and it was unclear if it was drug related or simply caused by the behavior of an untrained animal (Devine et al., 2013). Most opioids have minimal effects on cardiac output in dogs, with clinically relevant doses resulting in changes ranging from slight increases to slight decreases, but methadone may produce more consistent decreases in cardiac output (Buckhold et al., 1977; Priano and Vatner, 1981; Copland et al., 1987; Maiante et al., 2009). Opioids produce bradycardia in dogs, but they usually compensate well to maintain cardiac output and treatment for the bradycardia is not routinely needed (Copland et al., 1992). Only if necessary should bradycardia can be reversed with an anticholinergic (e.g., atropine). Cardiac output is also maintained with opioids in horses and may be increased if excitatory effects occur (Muir et al., 1978; Robertson et al., 1981; Carregaro et al., 2006). Opioids produce minimal vascular effects when administered to animals in clinically relevant doses (Barnhart et al., 2000; Pant et al., 1983; Hug et al., 1981; Kayaalp and Kaymakcalan, 1966; Priano and Vatner, 1981; Carregaro et al., 2006). Changes in mean arterial pressure range from minimal increases to minimal decreases (∼10% from baseline values). Increases in plasma histamine concentrations following morphine administration are often cited as producing hypotensive effects in dogs, but histamine release is short lived (Guedes et al., 2007a) and minimal effects on blood pressure are noted following IV morphine administration. The cardiovascular effects of opioids appear to be more pronounced in anesthetized animals due to interactions with the anesthetic and when supratherapeutic opioid are administered. The effects of opioids on myocardial blood flow are variable with some studies demonstrating increases in blood flow where others show decreases, but these studies assessed supratherapeutic doses, ≥2 mg/kg (Pant et al., 1983; Vatner et al., 1975). This is in contrast to the consistent effects in humans, coronary vasodilation and increased myocardial perfusion. Morphine’s effect on vascular tone has been used in the past as a treatment for acute cardiogenic pulmonary edema (Vismara et al., 1976). The benefits are attributed to splanchnic blood pooling, afterload reduction, or reduced breathing effort. Some investigators attributed the benefits to vasodilation caused by histamine release. This is considered a rather outdated use of morphine. Today we have potent diuretics and vasodilators to treat this condition. Opioids produce an antitussive effect through a central inhibition of the cough center independent of their respiratory depressant effects (Chou and Wang, 1975). Both μ- and κ- receptor agonists have been documented to produce antitussive effects (Takahama and Shirasaki, 2007). The antitussive effect is more resistant to naloxone reversal than the analgesic effect of opioids (Chau et al., 1983). Morphine, codeine, butorphanol, and methadone have demonstrated antitussive effects in dogs or cats, and tramadol additionally in cats (Rosiere et al., 1956; Cavanagh et al., 1976; Nosal’ova et al., 1991). Hydrocodone has antitussive properties in rats and people, and is commonly used in dogs for this purpose although studies definitively demonstrating its efficacy in dogs and cats are lacking (Kasé et al., 1959; Hennies et al., 1988; Homsi et al., 2002). Dextromethorphan, which does not affect opiate receptors, is used as an over-the-counter medication to treat coughing in people. It is believed to bind to high- and low-affinity sites in the brain that are distinct from opiate receptors (Grattan et al., 1995). A steric hindrance may prevent binding to the typical opiate receptors responsible for analgesic and other effects. In people, it is more effective in spontaneous naturally occurring cough than in experimental models (Capon et al., 1996). Dextromethorphan has been shown to be an effective antitussive in dogs after IV injection (ED50 = 10.2 mg/kg IV), but the oral bioavailability is questionable (discussed more specifically later in Section Clinical Pharmacology, Antitussive) (Kasé et al., 1959; KuKanich and Papich, 2004a). Because it is believed that the parent drug, dextromethorphan, rather than a metabolite, is responsible for the antitussive effect (Capon et al., 1996), it is unlikely that oral administration will be effective as an antitussive in dogs. Emesis and nausea can occur following administration of opioids via stimulation of the chemoreceptor trigger zone (CRTZ) (Mitchelson, 1992). Opioids also may stimulate central dopamine (D2) receptors in the vomiting center to produce vomiting. Opioids appear to have differing emetic effects, with morphine causing emesis more frequently than hydromorphone or oxymorphone in dogs (Valverde et al., 2004). The emetic response is complicated by the observation that opiates also act as antiemetics in the vomiting center (Scherkl et al., 1990), with an antiemetic effect predominant for butorphanol, methadone, and fentanyl (Schurig et al., 1982; Blancquaert et al., 1986). It is not unusual to observe emesis after an initial dose of morphine or hydromorphone to a patient, but not with subsequent doses. Apomorphine administered initially will produce reliable emesis in dogs, subsequent doses do not (Khan et al., 2012). Apomorphine is discussed in more detail in Section Opioid Agonists, Apomorphine. It is commonly observed that vomiting occurs after morphine administration to pain-free animals (e.g., premedication, research animals), but vomiting is not commonly observed in animals with moderate to severe pain (e.g., trauma). These observations may be related to the antiemetic effects produced after an initial dose, the antiemetic effects produced by endogenous opioids in painful patients, or mitigation of emesis by pain. Since the CRTZ is not protected by the blood–brain barrier, low plasma concentrations can produce emesis following oral morphine administration without producing an analgesic effect (Blancquaert et al., 1986; KuKanich et al., 2005a). In cats, morphine appears to produce more of an emetic effect as compared to fentanyl, meperidine, buprenorphine, and butorphanol (Taylor et al., 2001; Robertson et al., 2003; Robertson et al., 2005). Hydromorphone produces signs of nausea (salivation and lip licking) in cats and may elicit emesis (Wegner et al., 2004). Release of histamine or dopaminergic effects also may produce emesis. Apomorphine, one of the most reliable emetics in dogs, is a central-acting dopamine (DA1, DA2) agonist. Dopamine is one of the neurotransmitters in the vomiting center as well as in the CRTZ. Likewise, histamine is released for a short time after morphine administration to dogs (Guedes et al., 2007a) and histamine is also known as one of the neurotransmitters involved in emesis. Opioids produce a decrease in gastrointestinal motility via both central and peripheral mechanisms. Opioid-mediated effects in the gastrointestinal tract are through the μ, κ, and δ opiate receptors. The review by DeHaven-Hudkins et al. (2008) provided details on the distribution of the receptors in the gastrointestinal tract and effects associated with stimulation, either from endogenous opioids or exogenous opioids. Opioid receptor-mediated stimulation of the gastrointestinal tract slows gastric emptying, decreases fluid secretion and increases intestinal fluid absorption, reduces propulsive motility, and increases pyloric and other sphincter tone. Expression of μ opiate receptors have been found in the submucosal plexus, myenteric plexus, and longitudinal muscle of the ileum. In healthy animals, the regulation of these receptors and their function helps to maintain gut homeostasis within the enteric nervous system by coordinating intestinal motility and intestinal secretions. Motility-induced changes by the μ, κ, and δ opiate receptors are accomplished by inhibiting Ca2+ channels decreasing the release of intestinal acetylcholine (Ach) and/or substance P (Cherubini et al., 1985; Galligan and Akbarali, 2014). The μ and δ opiate receptors also result in membrane hyperpolarization and decreased cAMP and protein kinase A (PKA) activity, resulting in reduced neuronal excitability. The μ and δ opiate receptors decrease intestinal fluid secretion by inhibition of chloride secretion and passive water movement into the colon. The clinical consequence is constipation or antidiarrheal effects. After the initial emetic effect, opioids decrease gastric motility, resulting in prolonged gastric emptying. Opioids can also increase the tone of the antrum and the duodenum resulting in difficult intubation with an endoscope. The small intestinal effects of opioids initially appeared to be limited to the proximal portions; however, additional studies demonstrated effects on the ileum as well. Decreased propulsive motility and decreased intestinal, pancreatic, and biliary secretions occur in the small intestine. There is increased tone of all gastrointestinal sphincters, including those of the pancreatic duct. However, increases in rhythmic, segmental, nonpropulsive contractions also occur, which enhances reabsorption of water. Morphine initially stimulates large bowel motility and defecation often occurs shortly after administration in dogs and cats (Tuttle and Elliot, 1969; Barnhart et al., 2000; Lucas et al., 2001). Following initial defecation, opioids produce a decrease in colonic propulsive motility and secretions, but increases in nonpropulsive rhythmic contractions occur similar to that in the small intestine. Therefore the passage of colonic contents is delayed and the fluid content is decreased. The decreased gastrointestinal motility can result in constipation, an adverse effect, or can be used therapeutically as a treatment for diarrhea. The decrease in intestinal motility is profoundly important for horses. Horses treated for pain are often prone to ileus. Administration of opioids to horses will decrease production of feces, decrease intestinal sounds, and potentially lead to ileus and constipation (Boscan et al., 2006). The effects are best known for morphine, but also can occur with butorphanol, an agonist/antagonist (Sellon et al., 2001). The butorphanol effects are dependent on method of administration because when butorphanol was administered by a constant rate infusion, the intestinal effects were less compared to an IV bolus injection (Sellon et al., 2001). Opioids produce a variety of effects on the urinary tract. μ-agonists increase the tone of urinary sphincters. Micturition is inhibited, which may result in urine retention and may be mediated by spinal as opposed to local mechanisms (Drenger et al., 1986, 1989). Mu-receptor agonists decrease urine production. As discussed by Robertson et al. (2001), although it has been documented that morphine produces an antidiuretic effect and decrease in urine output, the exact mechanism is unclear. It is not known whether it is caused by a release of arginine vasopressin (AVP) (also known as antidiuretic hormone), or via some other mechanism. In contrast, κ opioid agonists produce a diuretic effect as a result of decreased AVP concentrations (Craft et al., 2000). For a drug such as morphine that acts as an agonist for both receptors, the net effect observed clinically is diuresis in most situations. This is complicated by the effect on the urinary bladder. Morphine increases the tone of the sphincter of the urinary bladder, making it more difficult for some animals to voluntarily urinate. Morphine also inhibits the urinary voiding reflex. As a result of these effects hospitalized animals should be monitored and interventions used to facilitate bladder emptying when necessary. The effects of opioids on the immune system are complex. The immune system effects are likely related to the specific opioid administered, dose, and the duration of treatment. A complex interaction of the immune system, sympathetic nervous system, endocrine system (hypothalamic–pituitary–adrenal axis), and direct effects on leukocytes occur. Immunosuppressive and immuostimulant properties have been identified depending on the experimental conditions. Some painful patients may benefit from opiate effects on immune function (Page and Ben-Eliyahu, 1997). Extrapolations of the effects of opioids on the immune system from studies on human opioid abusers are likely inaccurate for veterinary patients because studies in humans are in the context of high dose administration, chronic use, and tolerance of human opioid abusers. Although these traits are often associated with opioid use in humans, they have been well documented in animals. Tolerance and dependence can be demonstrated in animals, but opioids are seldom used for a long-enough period of time at large-enough dosages in veterinary patients for this issue to have clinical consequences. The NMDA receptor may play a role in opioid tolerance and dependence as treatment with NMDA antagonists attenuate withdrawal symptoms in experimental settings (Yeh et al., 2002). The consequence of tolerance is that drug dosages may need to be increased with chronic administration of opioids. Opioids administered for a period as short as 5–7 days may result in dependence in dogs. In one study in dogs, constant rate infusion to dogs at a rate of 1–5 mg/kg/day produced physical dependence by day 8 (Yoshimura et al., 1993). Withdrawal signs can be elicited in dogs after dependence with injections of naloxone. Signs of withdrawal in dogs include nausea, aggression, vocalization, vomiting, hyperactivity, hyperthermia, tremors, and salivation. Withdrawal can also be elicited from administration of a μ antagonist or partial agonist such as butorphanol or buprenorphine in opioid-dependent dogs (Yoshimura et al., 1993). Therefore, if an animal has been receiving continuous opioids drugs for longer than 5–7 days, withdrawal signs may occur after administration of an antagonist or partial agonist. However, clinical experience suggests that discontinuing the opioid rarely causes withdrawal signs for durations of administration less than a week. In contrast, withdrawal signs have occurred clinically when butorphanol (μ-antagonist) was administered to a dog after 1 week of morphine administration. Decreasing the dose of the μ agonist to wean a patient off an opioid or decrease opioid effects is a better strategy than switching to a partial agonist or antagonist. Opioids tend to be well absorbed when administered orally, intramuscularly, or subcutaneously. However, because of substantial first-pass metabolism the oral administration of most opioids results in poor bioavailability and erratic plasma concentrations in animals. Administration of opioids per rectum to animals result in a minimal increase in bioavailability over oral administration, since the majority of drug absorbed by this route of administration is still subject to first-pass hepatic metabolism due to uptake into the portal vein. Nasal and oral transmucosal administration of opioids can bypass first-pass hepatic metabolism. However irritation, the volume of drug to be delivered, dosing frequency, and species specific differences have limited the clinical effectiveness of transmucosal administration to buprenorphine in cats. Opioids are well distributed throughout the body. Opioids are bound to plasma proteins in variable amounts from low to high protein binding depending on the individual drug. The opioids are lipophilic and weak bases. A comparison of the lipophilicity, designated by the octanol/water partition coefficient, is shown in Table 13.6. The higher the partition coefficient, the greater is the lipophilicity. All are weak bases (some have acidic groups also) with pKa values above 8. This indicates that lipophilicity increases at pH values of 8 and higher. These physiochemical properties favor intracellular accumulation. Consequently, opioids have a large volume of distribution, which greatly exceeds total body water. Additionally, administration of opioids with high lipophilicity, such as fentanyl (the highest Log P in Table 13.6 ), can produce rapid redistribution from tissues that are highly perfused, such as the central nervous system, to tissues that are less perfused, such as muscle and fat. The result is a more rapid decrease in concentrations (and effect) in the target organs in comparison to total body elimination. Repeated doses or a constant rate infusion may result in drug accumulation with an increased effect and slower than expected recovery. This phenomenon is similar to that seen with repeated doses of thiobarbiturates. Table 13.6 Chemical properties of opioids aLogP, log of the octanol/water partition coefficient. Systemic clearance of most opioids in animals is high. As seen in Table 13.5, clearance approaches or exceeds hepatic blood flow for most drugs, designating these as high clearance drugs. Most opioids are metabolized to more polar compounds and subsequently excreted in the urine or bile, but a small amount of unchanged drug may be eliminated in the urine and feces. Conjugation reactions are the primary means for some opioid metabolism (e.g., morphine and codeine), although cytochrome P450 (CYP) mediated metabolism can also occur (e.g., fentanyl, methadone), which are compound and species specific. Some of the conjugated products (e.g., glucuronide conjugates of opiates) are pharmacologically active, but because of their higher polarity may not cross the blood–brain barrier. Extrahepatic metabolism of morphine occurs in dogs, resulting in clearance rates exceeding the combined hepatic blood flow and glomerular filtration rates. Glucuronide conjugation is the primary conjugation pathway of morphine in most animal species except cats, which are a species deficient in some glucuronidation mechanisms (Court and Greenblatt, 1997). Sulfate conjugation is the primary means of morphine metabolism in cats. Despite differences in metabolic pathways, cats effectively metabolize morphine with total body clearance approximating the expected hepatic blood flow rate indicative of a high extraction drug (Table 13.5 ). Oxidative metabolism, mediated by CYP, appears to be the primary mechanism of fentanyl (and derivatives), methadone, and meperidine metabolism. Remifentanil is metabolized by tissue and plasma esterases and produces an extremely short half-life. The pharmacokinetic parameters of opioids in dogs and cats are presented in Table 13.5 . Opioids are commonly used for analgesia and to relieve pain and anxiety from painful syndromes. Clinically, it is observed that there is great variability in response among patients, and between drugs. High variability has been recorded for pharmacokinetics in a species and analgesic responses varied by many fold in experimental studies. Therefore, careful observation and a willingness to adjust doses (or switch drugs) in individual patients is important to successful pain management. Severe pain, often associated with trauma, should be treated with μ opioids agonists with high intrinsic activity (e.g., morphine, hydromorphone, fentanyl, methadone). Although some references have stated opioids should not be administered to painful animals in shock, there is no basis for this recommendation. Pain can contribute markedly to the adverse and life-threatening effects associated with shock and opioids often improve the hemodynamics and tissue perfusion of painful patients. As previously stated, the hypotensive effects of intravenous opioids (including morphine) are minimal when administered in clinically relevant doses, and concurrent supportive measures, such as fluid therapy, are essential to successful therapy in these patients. Mild to moderate pain (e.g., soft tissue surgery) has been managed with μ opioid agonists, partial agonists, mixed agonist–antagonists, or tramadol. The efficacy (and lack thereof) and variability of oral tramadol are discussed later. Preemptive analgesia, dose administration prior surgical or a painful stimulus and continued into the postoperative period, provides more effective analgesia than holding off administration of opioids to the postoperative period in dogs (Lascelles et al., 1997). This is thought to occur by decreasing central sensitization (also known as the wind-up phenomenon). Chronic and neuropathic pain appear to be less responsive to opioid analgesia. Higher doses of opioids in humans are needed for controlling neuropathic pain (Rowbotham et al., 2003). For neuropathic pain, it may be more effective to use a multimodal approach utilizing opioids in combination with other drug classes such as NMDA antagonists (e.g., ketamine), gabapentin, local anesthetics (e.g., lidocaine), or α-2 agonists (e.g., dexmedetomidine) appear to be more effective in refractory cases. Butorphanol tablets (Torbutrol) are approved by the FDA for antitussive use in dogs. Hydrocodone is also routinely prescribed for its antitussive effects in dogs (see Chapter 48). Codeine exhibits poor bioavailability in dogs and may not be effective when administered orally. (Codeine has questionable antitussive properties in people as well (Takahama and Shirasaki, 2007).) Tramadol tablets have demonstrated effectiveness as an antitussive in experimental models in cats due to the formation of an opioid metabolite, but have yet to be evaluated clinically. All opioid agonists are expected to be effective antitussives when administered parenterally. As mentioned previously, oral dextromethorphan – a nonopiate that is a common ingredient in human over-the-counter cough medications – is probably not an effective antitussive in dogs because of the low oral bioavailability and lack of activity from metabolites. As mentioned Section Opioid Pharmacodynamics, Cardiovascular Effects, routine use of morphine is no longer recommended in treatment of congestive heart failure in animals due to variable effects on coronary blood flow. Likewise, although used in the past as a treatment for acute cardiogenic pulmonary edema (Vismara et al., 1976), this is no longer a common use. Opioids are effective antidiarrheal drugs as they decrease gastrointestinal secretions, decrease propulsive gastrointestinal contractions, and increase nonpropulsive segmental gastrointestinal contractions (see Chapter 46). Loperamide is the most commonly recommended opioid for the treatment of diarrhea because of reliable effects, availability over the counter (not a controlled substance), low cost, and safety profile. Loperamide is p-glycoprotein (p-gp) substrate and as a result is excluded from the CNS in normal animals. However, if concurrent p-gp inhibitors (e.g., cyclosporine, ketoconazole, spinosad) are coadministered or the animal is deficient in p-gp (some Collie dogs, Collie related breeds, and other herding breeds) than sedation, dysphoria, and other marked CNS opioid effects may be noted for prolonged periods of time, which may exceed 24 hours (Hugnet et al., 1996). Diphenoxylate in combination with atropine is also marketed as an antidiarrheal for humans, but is not used frequently in veterinary medicine as it has not demonstrated increased efficacy over loperamide. Diphenoxylate is a prescription drug, and is a schedule V controlled substance requiring special record keeping. Opioids are commonly coadministered with sedatives, tranquilizers, and anesthetics. Additive or synergistic sedative and analgesic effects occur when opioids are used in combination with sedatives and anesthetics. Combinations of these drug classes result in decreased dosages of the individual drugs with the potential for decreased adverse effects. Additionally, preemptive analgesia increases the effectiveness of postoperative analgesics, decreasing opioid consumption, adverse effects, and cost. A more thorough review of sedation and anesthesia drug combinations is presented in Chapters 12 and 14. As a drug class opioids exhibit a wide safety margin. Cautious use of opioids in patients with head trauma is warranted as respiratory depression may increase blood carbon dioxide concentrations resulting in cerebral vasodilation and worsening effects of cerebral edema. However, use of lower doses more frequently or constant rate infusions will mitigate some of the adverse effects as they are concentration (peak) proportional. Opioids have varying effects on temperature regulation depending on the species (Adler et al., 1988). Panting occurs after administration of some opioids in some dogs. Hydromorphone and oxymorphone caused 60% of the dogs to pant in one study (Smith et al., 2001). Panting can be aggravating, especially in animals being induced with inhalant anesthetics or undergoing diagnostic procedures such as radiography or ultrasound, but is usually self-limiting. It is not related to effects on the respiratory center, but is a reaction to the effect of opioids on the thermoregulatory center of the hypothalamus, whereby the body’s set point is typically decreased by 1–3°F and dogs pant to lower their temperature. The depression of a dog’s body temperature is a consistent finding in published studies (Lucas et al., 2001). In contrast, administration of opioids has produced increase in body temperature in cats, horses, cattle, swine, and goats. Hydromorphone has been the best documented to produce this reaction, but other opioids are also associated with hyperthermia including morphine, buprenorphine, and butorphanol (Posner et al., 2007; Niedfeldt and Robertson, 2006). In published studies hyperthermia associated with hydromorphone has been as high as 41–42°C (107–108°F) in cats, for as long as 5 hours. The authors of these studies warn of high temperatures affecting postoperative recovery in cats when opioids are used for analgesia or perioperative sedation. There has been a controversy as to whether or not administration of opioid pure agonists with opioid agonist/antagonists will produce an interaction that diminishes the analgesic effect of the combination. There is a possibility that drugs such as butorphanol and pentazocine, which have antagonistic properties on the μ receptor, will partially reverse some effects of pure agonists such as morphine if they are administered together. The clinical relevance of this antagonism has been debated. There are few reports that have documented that such a combination is antagonistic. Reversal of the pure μ opioids can occur and acute pain or signs of opioid withdrawal may be precipitated following concurrent administration (Lascelles and Robertson, 2004). One use of antagonistic qualities of the opioids has been to administer a μ-receptor antagonist, or partial agonist (e.g., buprenorphine) to lessen some of the dysphoric effects caused occasionally from pure μ-receptor agonists such as morphine or fentanyl, while still providing some degree of analgesia. In dogs, butorphanol can partially reverse the effects of oxymorphone (Lemke et al., 1996). Butorphanol may reverse some respiratory depression and sedation from pure agonists, but some of the analgesic efficacy is preserved. In dogs that received butorphanol for postoperative pain associated with orthopedic surgery, there was no diminished efficacy from subsequent administration of oxymorphone (Pibarot et al., 1997). However, in another study, some dogs that had not responded to butorphanol after shoulder arthrotomy responded to subsequent administration of oxymorphone, but as expected the oxymorphone dose required to produce an adequate effect was higher than what would be required if oxymorphone was used alone (Mathews et al., 1996). Other results have been observed in cats. Increased analgesia, decreased analgesia, or no effect has been observed from combinations of agonists and antagonists. When butorphanol and oxymorphone were administered to cats in combination, there was greater efficacy than either drug used alone, with no observed antagonistic effects (Sawyer et al., 1994; Briggs et al., 1998). On the other hand, the administration of hydromorphone with butorphanol may decrease the analgesic from either drug used alone (Lascelles and Robertson, 2004). When butorphanol, buprenorphine, and the combination of both drugs were administered to cats all three treatments provided similar antinociceptive effects (Johnson et al., 2007). Although butorphanol is a κ-receptor agonist/μ-receptor antagonist, and buprenorphine is a κ-receptor antagonist/μ-receptor partial agonist, there was no antagonism detected. However, the design of the study may have prevented a more sensitive delineation of treatment effects. In general, administration of combinations of agonists and antagonist is not recommended due to variable and potential antagonistic effect. There are data available in the human literature documenting enhanced analgesic effects and reduced tolerance by coadministering ultra-low-dose opioid antagonists with μ-agonists. The doses of the antagonists are lower than needed to completely antagonize the agonist. For example, naloxone administered as a constant rate infusion (0.25 μg/kg/h) significantly reduced the morphine consumption and gastrointestinal adverse effects in hysterectomy patients (Movafegh et al., 2012). Even lower doses have been examined in experimental animals and humans (la Vincente et al., 2008). In these studies an “ultralow naloxone” dose is in the range of 3.5 μg total dose for a 70 kg human (0.05 μg/kg). When combined with an opioid, these low doses produced significantly greater enhanced analgesia with fewer adverse effects than when the opioid was administered alone. This is in contrast to the typical naloxone dose of 0.4 mg total dose to antagonize opioid agonists in humans. Although this is an area worthy of further study in veterinary medicine, optimized doses, drug combinations, and demonstrated benefits in veterinary species are lacking. Opioids are high clearance drugs in animals; therefore, they rely more on hepatic blood flow than activity of liver enzymes for clearance. Diseases or conditions that impair hepatic blood flow (e.g., cardiac disease, hypotension, hypovolemia) may substantially affect opioid drug clearance. Interactions with hepatic microsomal cytochrome P450 enzymes (CYP), protein binding, or loss of hepatic function are not expected to affect most opioids’ drug clearance, but some exceptions exist. The pharmacokinetics of methadone are significantly affected when combined with chloramphenicol (KuKanich and KuKanich, 2015), resulting in prolonged methadone drug exposure, higher maximum concentrations and pronounced opioid effects. The effects of chloramphenicol on other opioids likely metabolized by CYP (e.g., fentanyl, meperidine) have not been reported, but interactions could occur. An opioid not metabolized by CYP (e.g., morphine) would be a better choice until more data are available. Renal impairment decreases the clearance of morphine glucuronide metabolites with a resultant metabolite accumulation. However, the importance of opioid glucuronide metabolites has not been demonstrated in animals and clinical consequence of metabolite accumulation has not been demonstrated in animals. The most common drug–drug interactions with opioids occur when combined with tranquilizers/sedatives and is often used clinically to increase sedation. There is a specific interaction described in people between monoamine oxidase (MAO) inhibitors and meperidine. The interaction has been most commonly observed with older nonselective and irreversible inhibitors of MAO. The use of these drugs together has caused an unpredictable, and a sometimes fatal reaction. The reaction includes excitation, sweating, rigidity, coma, and seizures. This reaction is due to the effect of meperidine (and its metabolite normeperidine) on serotonin turnover in conjunction with the MAO inhibitors decreasing metabolism of serotonin, resulting in serotonin syndrome. This is discussed in more detail later in Section Opioid Agonists, Meperidine(Pethidine). Other analgesics that may be affected include methadone and tramadol as they also both affect serotonin turnover. The interaction with opioids that do not affect serotonin turnover (e.g., morphine, hydromorphone, fentanyl, butorphanol, etc.) is unlikely. It has been suggested if an animal is receiving an MAO inhibitor, that a test dose of the opioid should be administered. If there is no adverse reaction, subsequent doses can probably be administered safely. Although nonselective MAO inhibitors (e.g., type A and B MAO inhibitors) are rarely used in veterinary medicine for treatment of depression as they are in people, other drugs with MAO-inhibiting qualities are used in animals. For example, selegiline, a specific MAO-type B inhibitor (deprenyl, Anipryl), is used in dogs to treat canine hyperadrenocorticism and cognitive disorder (discussed in Chapter 18). A study assessing the effects of selegiline on oxymorphone and butorphanol in dogs failed to demonstrate a detrimental interaction (Dodam et al., 2004). Amitraz (Mitaban) is also a MAO-inhibitor and is found in pet collars and dips to prevent and treat mite infections in animals (see Chapter 43). Although no reactions in animals have been described between amitraz or selegiline and opioid analgesic drugs, one should be aware of a potential interaction and the animal monitored closely for adverse effects. Use of other opioids in food producing animals is permitted by the Animal Medicinal Drug Use Clarification Act (AMDUCA) if an adequate withdrawal interval can be determined. Therefore the Food Animal Residue Avoidance Databank (FARAD) or a reliable reference (Papich, 1996, 2016) should be consulted prior to opioid administration to food producing species in order to obtain the most current withdrawal interval recommendations. Morphine is commonly used in veterinary medicine due to its safety, efficacy, tolerability, and low cost, despite the development of newer opioids. Current dosage recommendations are presented in Table 13.7. Morphine is effective for mild to severely painful conditions. Intravenous administration results in dose-dependent increases in plasma histamine (Thompson and Walton, 1964), but at doses <0.6 mg/kg IV, no significant changes occurred in blood pressure in awake dogs (Guedes et al., 2006, 2007a). Higher doses, 3 mg/kg IV, resulted in higher plasma histamine and significant decreases in blood pressure in pentobarbital anesthetized dogs (Muldoon et al., 1984). Histamine release may possibly account for transient excitement, but it is short lived (Robinson et al., 1988; Guedes et al., 2007a). Morphine exhibits moderate protein binding in most species approximating 30%. Morphine is also produced endogenously in both humans and animals in response to inflammation and stress (Brix-Christensen et al., 2000; Yoshida et al., 2000). Table 13.7 Morphine dosages (only parenteral doses are listed, oral administration is not recommended) NR, not recommended. Even though the clinical morphine dose is much smaller (Table 13.7 ), at a dose of 3 mg/kg it showed a wide safety profile in dogs. At high doses of 40 mg/kg it produced severe coronary perfusion effects, 180 mg/kg eliciting seizures in ventilated dogs, and death at doses higher than 200 mg/kg in dogs (de Castro et al., 1979; Muldoon et al., 1984). The reported IV LD50 of morphine (HCl) in dogs is 175 mg/kg (Kasé et al., 1959). The pharmacokinetics of morphine were reported in several studies and are summarized in Table 13.5. Constant rate IV infusion of morphine in dog studies demonstrate the high clearance rates and need for high infusion rates (KuKanich et al., 2005c; Lucas et al., 2001; Guedes et al., 2007b). Because of its low oral and rectal bioavailability, morphine should be administered parenterally in order to consistently achieve therapeutic concentrations. The low lipophilicity in comparison to other opioids (Table 13.6
Opioid Analgesic Drugs
Introduction
Analgesia (antinociception)
Sedation
Calming and euphoria
Immobilization and chemical restraint
Diarrhea, inhibition of gastrointestinal motility
Antitussive
Adjunctive use for general anesthesia
Increased locomotor activity (used illegally in performance horses for this purpose)
Background on Opioids
Opioid Role in Analgesia
History of Opioids
Substance
DEA number
Other names and brand names
Schedule I
Acetyldihydrocodeine
9051
Acetylcodone
Benzylmorphine
9052
Codeine methylbromide
9070
Codeine-N-oxide
9053
Desomorphine
9055
Dihydromorphine
9145
Etorphine (except HCl)
9056
Heroin
9200
Diacetylmorphine, diamorphine
Morphine methylbromide
9305
Morphine methylsulfonate
9306
Morphine-N-oxide
9307
Nicomorphine
9312
Vilan
Normorphine
9313
Schedule II
Alfentanil
9737
Alfenta
Carfentanil
9743
Wildnil
Dihydrocodeine
9120
Didrate, Parzone
Diphenoxylate
9170
Diprenorphine
9058
M50-50
Ethylmorphine
9190
Dionin
Etorphine HCl
9059
M 99
Fentanyl
9801
Innovar, Sublimaze, Duragesic
Hydrocodone
9193
dihydrocodeinone
Hydrocodone and isoquinoline alkaloid 15 mg/du
9805
Dihydrocodeinone, papaverine or noscapine
Hydrocodone combination product 15 mg/du
9806
Tussionex, Tussend, Lortab, Vicodin, Hycodan, Anexsia
Hydromorphone
9150
Dilaudid, dihydromorphinone
Levomethorphan
9210
Levorphanol
9220
Levo-Dromoran
Meperidine
9230
Demerol, Mepergan, pethidine
Methadone
9250
Dolophine, Methadose, Amidone
Methadone intermediate
9254
Methadone precursor
Morphine
9300
MS Contin, Roxanol, Duramorph, RMS, MSIR
Opium extracts
9610
Opium fluid extract
9620
Opium poppy
9650
Papaver somniferum
Opium tincture
9630
Laudanum
Opium, granulated
9640
Granulated opium
Opium, powdered
9639
Powdered Opium
Opium, raw
9600
Raw opium, gum opium
Oxycodone
9143
OxyContin, Percocet, Tylox, Roxicodone, Roxicet,
Oxymorphone
9652
Numorphan
Racemethorphan
9732
Racemorphan
9733
Dromoran
Remifentanil
9739
Ultiva
Sufentanil
9740
Sufenta
Schedule III
Buprenorphine
9064
Buprenex, Temgesic
Butabarbital
2100
Butisol, Butibel
Codeine combination product 90 mg/du
9804
Empirin, Fiorinal, Tylenol, ASA or APAP w/codeine
Nalorphine
9400
Nalline
Schedule IV
Butorphanol
9720
Stadol, Stadol NS, Torbugesic, Torbutrol
Pentazocine
9709
Talwin, Talwin NX, Talacen, Talwin Compound
Schedule V
Codeine preparations: 200 mg/100 ml or 100 g
Cosanyl, Robitussin A-C, Cheracol, Cerose, Pediacof
Dihydrocodeine preparations: 10 mg/100 ml or 100 g
Cophene-S, various others
Diphenoxylate preparations: 2.5 mg/25 μg atropine sulfate
Lomotil, Logen
Ethylmorphine preparations: 100 mg/100 ml or 100 g
Opium preparations: 100 mg/100 ml or 100 g
Parepectolin, Kapectolin PG, Kaolin Pectin P.G.
Physiology of Opioids
μ Receptor
κ Receptor
δ Receptor
Analgesia (spinal and supraspinal)
Respiratory depression
Decrease gastrointestinal motility
Decrease gastrointestinal secretions
Decrease biliary secretions
Increase appetite
Sedation/excitement (dose and species dependent)
Euphoria
Antidiuresis
Decrease urine voiding reflex
Decrease uterine contractions
Miosis/mydriasis (species specific)
Nausea/vomiting or antiemetic (drug specific)
Immunomodulation
Analgesia (spinal and supraspinal)
Decrease gastrointestinal motility
Decrease gastrointestinal secretions
Increase appetite
Sedation
Diuresis (decrease ADH release)
Miosis/mydriasis (species specific)
Nausea/vomiting or antiemetic (drug specific)
Analgesia (spinal and supraspinal)
Increase appetite
Immunomodulation
Opioid Pharmacodynamics
Analgesia
μ
κ
δ
α-2
5-HT
NMDA
M1
M3
GABA
Morphine
++
+
Hydromorphone
++
Oxymorphone
++
Hydrocodone
+
Codeine
+
Oxycodone
++
Heroin
++
+*
Methadone
++
+
–
Meperidine
++
++
–
Fentanyl
++
Carfentanil
++
Etorphine
++
++
++
Propoxyphene
+
Buprenorphine
+
Butorphanol
+
++
Tramadol
+**
+
++
–**
Nalbuphine
–
++
Pentazocine
+
++
Nalorphine
–
+
Naloxone
–
–
–
–
Naltrexone
–
–
–
Nalmefene
–
–
–
Diprenorphine
–
–
–
Respiratory Depression
Central Nervous System Excitation
Half-life
Clearance
Volume of
Oral
IV LD50
(h)
(ml/min/kg)
distribution (l/kg)
bioavailability
(mg/kg)
Dogs
Cats
Dogs
Cats
Dogs
Cats
Dogs
Cats
Dogs
Morphine
1.2
1.1–1.3
60
24, 42
4
2.6, 2.8
5–20 %
175
Oxymorphone
0.8
1.8
52
36.2
3.7
5.5
Hydromorphone
0.6–1.0
0.8–1.6
68–106
28–68
4.5–5.3
3.1–4.9
Fentanyl
3–6
∼2.5
35
20
10
2.6
14
Meperidine
0.8
1.8
43
40
1.9
4.0
11 %
68
Methadone
2–4
4
30
7.2
3.5
2.3
low
29
Alfentanil
0.33
0.4
29.8
11.6
0.6
0.9
>20
Remifentanil
0.09
48–63
0.2
Sufentanil
0.9
17.6
0.8
14
Oxycodone
1.9*
low*
Hydrocodone
1.7
41
5.0
40–84 %
Codeine
1.2–1.5
30–36
3.2
4–6.5 %
98
Buprenorphine
5
6.9
16
16
17.5
7.1
3–6 %
79
Butorphanol
1.6**
6.6**
57**
12.7**
8.0**
7.7**
10
Nalbuphine
1.2
46
4.6
5.6 %
140
Tramadol
0.8
2.2
55
21
3.0
3.0
4–65 %
93%
40–100
Cardiovascular Effects
Antitussive Effect
Gastrointestinal Effects
Emetic Properties
Gastrointestinal Motility
Urinary Tract
Immune System Effects
Tolerance and Dependence
Opioid Pharmacokinetics
Opiate
LogPa
Solubility (mg/l)
pKa
Meperidine
2.72
6550
8.59
Buprenorphine
4.98
0.0168
8.31
Butorphanol
3.3
0.16
10.7
Sufentanyl
3.95
76
8.86
Fentanyl
4.05
200
8.77
Methadone
3.93
48.5
8.94
Hydromorphone
0.9
4.39
8.59
Oxymorphone
0.83
2.4 × 104
8.17
Morphine
0.89
149
8.21
Clinical Pharmacology
Analgesia
Antitussive
Congestive Heart Failure
Antidiarrheal
Sedation Anesthesia
Contraindications, Warnings, and Drug Interactions
Head Trauma
Temperature Regulation
Interactions Between Opioid Drugs
Interactions with Drug Clearance
Interactions with Other Analgesics and Antidepressants
Uses In Food-Producing Animals
Opioid Agonists
Morphine
IV
IV infusion
IM/SC
Dog
0.25–0.5 mg/kg q 2–3 h
0.1–0.2 mg/kg/h
0.25–1 mg/kg q 2–4 h
Cat
0.1–0.25 mg/kg q 2–3 h
0.05–0.1 mg/kg/h
0.1–0.5 mg/kg q 2–4 h
Horse
0.1–0.2 mg/kg q 8 h
NR
0.1–0.2 mg/kg q 8 h
Sheep
≤ 10 mg total dose
Goat
≤ 10 mg total dose
Swine
0.2–0.9 mg/kg
Rat / mouse
2–5 mg/kg q 4 h
Guinea Pig / Hamster
2–5 mg/kg q 4 h
Rabbit
2–5 mg/kg q 4 h
Primates
0.5–2 mg/kg q 4–6 h
Ferrets
0.5 mg/kg q 4–6 h
Dogs
Stay updated, free articles. Join our Telegram channel
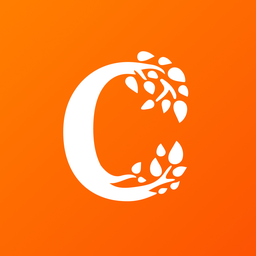
Full access? Get Clinical Tree
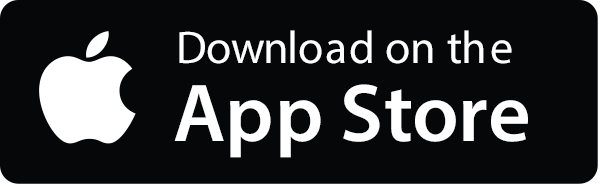
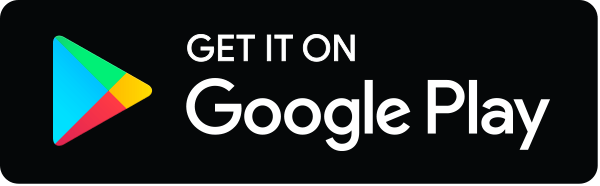