CHAPTER 23 David C. Dorman There are thousands of species of plants, household products, prescription drugs, and nonprescription drugs in the United States that could result in companion animal exposure. On occasion, serious poisonings, with or without neurologic signs, can occur following exposure to these agents. This chapter focuses on common poisons that are known to induce neurotoxicity in companion animals. Earlier editions of this textbook have included agents that remain of interest to veterinarians. These include 4-aminopyridine (Avitrol), cocaine, 5-fluorouracil (5-FU), lindane, and other organochlorine insecticides, penitrems, strychnine, sodium fluoroacetate (compound 1080), hexachlorophene, methionine, morphine, and the organophosphate insecticide chlorpyrifos, especially as it relates to organophosphate-induced delayed neuropathy (OPIDN). Unfortunately, no single document can adequately address even a small fraction of the potentially toxic agents that exist. An invaluable resource for veterinarians and clients remains the ASPCA’s National Animal Poison Control Center (www.napcc.aspca.org), which provides a fee-for-service, round-the-clock toxicology telephone “hotline” (900-680-0000 or 888-426-4435). The center can provide veterinarians with specific treatment protocols and toxicity data for many of these agents. Clinical signs (e.g. seizures) have been used to organize the chapter. This scheme is, however, simplistic because many neurotoxicants induce multiple effects, and veterinarians may miss observing critical phases of the toxic syndrome. For example, clinical signs in animals exposed to an organophosphate insecticide often include salivation, lacrimation, urination, and defecation (SLUD), miosis, bradycardia, muscle tremors, amongst others. However, some these signs (e.g. SLUD) often develop early in the course of the toxic syndrome and only a subset of these signs may be present when an animal is seen by the veterinarian. Neurologic signs can also develop due to effects on other organ systems. For example, severe hepatobiliary injury is often associated with hepatic encephalopathy. Differentiating primary from secondary neurotoxicity relies in part on the identification of additional body systems that are involved. The first diagnostic challenge often is to determine whether an animal has been poisoned (or is at risk of developing toxicosis if an exposure occurred). Three interrelated factors help establish a toxicological diagnosis: (1) history of exposure, (2) determination of whether the exposure dose is sufficient to induce toxicity, and (3) development of compatible clinical signs. Additional findings that may further support a diagnosis of primary neurotoxicosis include (1) an acute onset and/or rapid progression of neurologic signs in a previously normal animal, (2) neurologic signs in the absence of multiple organ system involvement, (3) more than one animal involved, and (4) multifocal involvement of the nervous system (i.e. mixed cranial nerve, peripheral nervous system [PNS], and neuromuscular involvement). In all cases, a presumptive diagnosis should not be based upon a single factor. Chemical analysis of tissues remains an important criterion and often serves as the “gold standard” for confirming a toxicological diagnosis. Freshly frozen postmortem tissue samples (1–10 g), including brain, liver, kidney, fat, GI contents, and skin (when dermal exposure occurred), should be collected for toxicological (chemical) analysis. Positive forensic findings are not always definitive proof of poisoning; conversely, negative findings don’t always rule out poisoning. Neurophysiological evaluations (e.g. electroencephalography [EEG], electromyography [EMG], evoked potentials) may occasionally provide ancillary clinical evidence consistent with poisoning. Alterations in peripheral blood samples are often nondiagnostic, but cerebrospinal fluid (CSF) analysis may be of use in certain instances. Macroscopic changes related to neurotoxicant exposure may include gross lesions or alterations in the size of a major brain region. Samples of brain, spinal cord (e.g. cervical and lumbar intumescences), and peripheral nerves (e.g. sciatic nerve) should be collected for histopathologic examinations from animals in which neurotoxicosis is suspected. Peripheral nerve tissue samples should be fixed while mildly stretched to prevent contraction artifacts. Morphologic lesions are often lacking in many neurotoxic syndromes, hence an absence of lesions can be as important diagnostically as their presence. In the veterinary clinical setting, samples are usually fixed by immersion in neutral buffered 10% formalin. Immersion-fixed neural tissues often exhibit artifacts (e.g. dark neurons, myelin bubbling, and neuropil vacuolation) associated with handling at necropsy and/or sub-optimal processing. In all cases, the careful correlation of data collected from the history, clinical and neurologic examinations, and supportive diagnostic tests is used to make a presumptive diagnosis of neurotoxicosis. Several reviews of the management of the poisoned dog and cat are available. The basic strategies used in the treatment of a poisoned animal are relatively straightforward and include (1) initiation of life support, (2) reducing the absorption of a chemical and/or enhancing its elimination, and (3) antagonism of pharmacologic effects. Gastrointestinal decontamination Gastrointestinal (GI) decontamination remains the most common therapeutic interventions used in clinical veterinary toxicology. GI decontamination is usually used following dermal exposure since oral exposure occurs in companion animals following grooming. The simplest method involves the administration of an emetic by an owner or veterinarian. Emesis is most productive if performed within 2–3 hrs post ingestion (earlier is better!). This timeframe is influenced by the exposure dose, presence of food in the GI tract, and the rate at which GI absorption occurs. Feeding the animal a small moist meal before inducing vomiting can increase the chances of an adequate emesis. Even with repeated emesis, use of an emetic generally removes only 40–60% of the stomach’s contents. Common contraindications for the use of emetics include (a) ingestion of a corrosive agent; (b) rodents, rabbits, horses, or other species incapable of safely vomiting; and (c) severe hypoxia, dyspnea, central nervous system (CNS) depression including coma, seizures, or pre-seizure activity, or other conditions that impair pharyngeal reflexes. Common emetics for home use include syrup of ipecac (dog: 1–2 mL/kg, cat: 3.3 mL/kg, PO) and 3% hydrogen peroxide (5–25 mL/5 kg, PO). Apomorphine (dog, 0.03 mg/kg, IV), and xylazine (cat, 0.44 mg/kg, IM, or SQ; dog, 1.1 mg/kg IM or SQ) can be used in a clinic setting. Apomorphine is a centrally acting emetic. Side effects such as CNS and respiratory depression, ataxia, excitement, and protracted vomiting can be seen with apomorphine. Many of these side effects can be reversed with naloxone (0.04 mg/kg IV, SQ, and IM.). Xylazine is an alpha 2-adrenergic agonist, which can cause bradycardia, hypotension, reduced respiratory rate, and CNS depression. The side effects of xylazine often outweigh the benefits for its use as an emetic. Yohimbine (0.1 mg/kg, IV) can be used to reverse xylazine. In some cases (e.g. potentially lethal oral exposures have occurred), gastric or enterogastric lavage under general anesthesia is indicated. Examples of neurotoxic agents when enterogastric lavage may be indicated would include strychnine, metaldehyde, tricyclic antidepressants, 5-fluorouracil, and isoniazid. Gastric lavage should not be performed in cases of caustic or petroleum distillate ingestion. Activated charcoal powder (1–4 g/kg, PO) combined with a saline (magnesium or sodium sulfate at 250 mg/kg, PO) or osmotic cathartic as a suspension in water (10 X volume) is also administered orally or by gastric tube. Unless otherwise indicated, the reader should assume that charcoal and a saline cathartic should be used for recent oral exposures (i.e. ingestion occurred within 1–2 hrs) to the agents discussed in the text. Control of seizures and CNS excitation Seizures and severe CNS excitation induced by a wide range of neurotoxicants are often controlled with diazepam (0.5 mg/kg, IV, repeated as needed every 10 min for up to three doses). Seizures may also initially be controlled with propofol (0.1 to 0.6 mg/kg/min, IV). The use of propofol requires establishing an airway and constant patient monitoring. Other seizure control medications (e.g. phenobarbital at 2.0 to 5.0 mg/kg, IV, q 20 min up to two times) may also be needed when diazepam or propofol fail to abolish seizure activity. As a rule, phenothiazine tranquilizers are best avoided in poisoned animals because they may aggravate CNS depression and, in some cases, may induce extrapyramidal effects, seizures, and hypotension. More detailed information on the management of seizures is provided in Chapter 9. Control of CNS or respiratory depression Naloxone is specifically recommended for the treatment of exogenous opiate (e.g. morphine, codeine) toxicosis because it lacks opiate agonist activity. The plasma half-life and duration of action of naloxone is relatively short (45 to 90 min), thus repeated doses (0.04 mg/kg, either IV, IM, or subcutaneous) are often required for the treatment of opiate-induced coma. Naloxone has also been used to manage nonopiate-induced CNS depression (i.e. from barbiturates and benzodiazepines). The value of analeptics (e.g. doxapram, nikethamide) in the treatment of poisoned animals is debatable at best, given that it is difficult to stabilize patients given these drugs. Potential adverse effects associated with doxapram include hypertension, arrhythmias, dyspnea, convulsions, and rebound CNS depression. Enhanced clearance following ingestion Increasing renal clearance through diuresis with or without ion trapping has limited application in neurotoxicology. One reason is that many neurotoxicants are highly fat-soluble and lipophilic agents are poorly excreted by the kidney without prior (hepatic) metabolism to more hydrophilic metabolites. In rare cases (e.g. amphetamine, phencyclidine), urine acidification with ammonium chloride (100 to 200 mg/kg/day, PO divided four times daily) or ascorbic acid (20 to 30 mg/kg, PO) is used to ion-trap weak organic bases. Urine acidification is, however, contraindicated if the animal has reduced renal function or if myoglobinuria is present (e.g. secondary to muscle damage). One emerging approach to enhancing whole body elimination is so-called lipid therapy. The administration of an intravenous lipid emulsion (ILE) was initially used in human medicine to manage local anesthetic systemic toxicity. The use of ILEs has subsequently shown promise as an effective antidote for other drug poisonings (e.g. ivermectin, moxidectin, verapamil). ILE is composed of neutral, medium to long-chain plant-based triglycerides. Formulations contain 10–30% lipid. The pharmacological mode of action of ILE remains unknown but may relate to beneficial metabolic effects and/or changing the lipophilicity of the blood resulting in the sequestration of fat-soluble materials in the blood (“lipid sink”). A recent in vitro study has shown that the binding of drugs to a lipid emulsion is favored when the drug is highly lipophilic (i.e. positive lipid partition constant) and has a large volume of distribution (i.e. the drug distributes into the fat and muscle). Potential adverse effects associated with ILE therapy include untoward drug–drug interaction (i.e. ILE may affect the efficacy of anticonvulsants and other therapies), pancreatitis, lipid emboli, product contamination (e.g. microbial growth if inappropriate handling or storage occurs), hypersensitivity reactions, and so-called fat overload syndrome, which is characterized by hyperlipidemia, hepatomegaly, icterus, and hemolysis. Lipemia secondary to ILE administration can also interfere with certain clinical chemistry tests (e.g. blood glucose). Several different ILE infusion protocols have been published. ILE therapy should use a 20% lipid solution that is given slowly as an intravenous infusion over 2–15 min. Typical loading (bolus) doses range from 1.5 to 2.0 mL/kg. A continuous rate infusion (CRI) at 0.06 to 0.5 mL/kg/min for 30–60 min is then used. Up to three bolus and CRI (total) infusions can be given. Repeated ILE administration should be considered if an animal remains symptomatic and the serum is clear of lipemia. ILE should not be repeated if the serum appears orange or yellow. The use of ILE therapy remains in its infancy in veterinary medicine and the ASPCA’s National Animal Poison Control Center has been at the forefront of this treatment approach. Consultation with this group prior to initiating ILE therapy is advised. Within this chapter, “seizures” refers to involuntary, paroxysmal brain disturbances usually manifested by uncontrollable muscular activity (e.g. paddling), abnormal psychomotor behavior (e.g. fly biting, tail chasing), autonomic dysfunction (e.g. urination, defecation, salivation), and altered behavior during the immediate postictal phase (e.g. CNS excitation, depression). Box 23.1 lists several poisons associated with CNS stimulation or seizures. Exposure to “seizurogenic” agents may result in hyperactivity, hyperesthesia, muscle tremors and fasciculations, and behavioral manifestations (e.g. aggression). Head trauma may result secondary to toxicant-induced ataxia and seizures. A recent review of cases presented to a German veterinary hospital revealed that toxicant exposure could account for approximately 4% of all cases of seizure disorders in dogs. Another recent United Kingdom study examining dogs with toxicant-induced status epilepticus showed that recurrent seizure episodes did not occur following prolonged (> 30 min) seizures. These results suggest that long-term treatment with antiepileptic drugs after the initial toxicant-induced seizure has been controlled isn’t warranted. Amphetamine and amphetamine derivatives Amphetamine poisoning in dogs and cats most commonly results from the accidental ingestion of amphetamine-based stimulants. These medications are used for the treatment of attention deficit hyperactivity disorder (ADHD) and/or narcolepsy. Examples of prescription amphetamine derivatives include Adderall (amphetamine and dextroamphetamine salts), Dexidrine (dextroamphetamine salts), and Desoxyn (methamphetamine hydrochloride). Amphetamine-based drugs are also increasingly used for recreational (i.e. illegal) purposes. The primary therapeutic action of amphetamines is monoamine (e.g. dopamine, norepinephrine) release. Amphetamine is chemically and structurally related to dopamine and noradrenaline and is a competitive substrate for the norepinephrine reuptake transporter (NET) and dopamine reuptake transporter (DAT). Amphetamine is actively transported into monoaminergic nerve terminals and induces impulse-independent release of cytosolic catecholamines. Since amphetamine’s isomers compete with endogenous catecholamines for transport into presynaptic terminals via NET and DAT, they delay synaptic neurotransmitter clearance. Amphetamine stimulates the release of catecholamines (e.g. norepinephrine) from the adrenal glands as well as the cerebral cortex, medullary respiratory center, and reticular activating system. Amphetamine poisoning may result in cardiac and CNS effects. Clinical signs develop within 1–2 hrs after ingestion and may include hyperactivity, mydriasis, hyperthermia, tachycardia, lactic acidosis, hypertension, and (infrequently) seizures. Hematological effects in dogs have also been reported and include a marked decrease in the number of nucleated red blood cells and leukogram abnormalities consistent with glucocorticoid-mediated stress. Amphetamine-induced hyperpyrexia with secondary bone marrow damage may account for the observed metarubricytosis. The amphetamine-like drug pemoline has largely been discontinued in the United States due to concerns related to hepatic toxicity. Pemoline has been shown to cause clinical signs in dogs at doses of > 2.8 mg/kg. The types of clinical signs detected in dogs with pemoline toxicosis are similar to those reported in dogs that ingested amphetamine and include agitation, tachycardia, hyperresponsiveness, hyperthermia, and mydriasis. Clinical signs and symptoms seen in children with pemoline toxicosis include sinus tachycardia, hypertension, hyperactivity, choreoathetoid movements, and hallucinations. The treatment of amphetamine toxicosis rests upon early GI decontamination and supportive care (including control of cardiac arrhythmias and hyperthermia). Modestly enhanced amphetamine elimination may occur following ion trapping with urine acidification. Chlorpromazine (10–18 mg/kg, IV) or haloperidol (1 mg/kg, IV) given after administration of a lethal intravenous dose of amphetamine sulfate (10 mg/kg) experimentally reduced the hyperthermia severity and also increased survival rates in amphetamine-poisoned dogs. Diazepam may also be used to control seizures and may assist in calming the affected animal. Although amphetamine poisoning is rarely recognized in animals, its true incidence may be higher because of the reluctance of owners to admit to illegal drug use. The reported estimated acute oral LD50 in rodents ranges from 10–30 mg/kg. Amphetamine may be detected in blood, CSF, and other tissue samples. Methylphenidate Methylphenidate is also used to control ADHD and narcolepsy in humans. Unlike the amphetamines, the pharmacologic mode of action of methylphenidate (as the d-isomer) is to inhibit noradrenaline and dopamine reuptake at the nerve terminus. Despite this difference, the pharmacodynamics of methylphenidate are similar to those of the amphetamines, with peak effects occurring 30–45 min after dosing. Immediate and extended release formulations of these drugs are available. The LD50 for methylphenidate in companion animals has not been established. An LD50 value of 190 mg/kg has been reported in mice. The reported mean dose of methylphenidate seen in clinically affected dogs referred to the ASPCA’s National Animal Poison Control Center was 14.1 mg/kg (lowest dose was 0.39 mg/kg). The most frequently reported clinical signs seen in companion animals following methylphenidate ingestion included agitation, hyperactivity, pacing, clinging, inappropriate urination, circling, excitation, lethargy, anxiety, muscle fasciculations and tremors, CNS depression, and ataxia. The cardiopulmonary and digestive systems are also frequently affected in exposed dogs. Additional clinical signs and symptoms reported with methylphenidate overdose in humans include hallucinations, seizures, tachycardia, cardiac arrhythmias, hypertension, and hyperthermia. Oral administration of methylphenidate results in rapid and nearly complete GI absorption; therefore, rapid GI decontamination is required (especially with the immediate release formulation). Control of cardiac arrhythmias, and hyperthermia, and the judicious correction of acid-base and electrolyte disturbances may also be required. Chlorpromazine (3 mg/kg, IV) or acepromazine (0.025 to 0.2 mg/kg, IV, IM, or SC) may be used to control hyperactivity, tremors, and other pacing, excitement, and CNS abnormalities. Propranolol (0.02 to 1.0 mg/kg, IV or 0.1 to 0.2 mg/kg, PO, q 8 hrs) may be used to control tachycardia and certain other cardiac arrhythmias. Caffeine The methylxanthine caffeine can be found in chocolate, coffee, and tea. Energy drinks and other similar products also contain appreciable quantities of caffeine. Drinks made from the fruit of the guarana (Paullinia cupana) plant can also contain high levels of caffeine. Clinical signs of caffeine toxicosis in dogs and cats generally develop within several hours of ingestion and may include vomiting (often the first sign), restlessness, hyperactivity, ataxia, muscle tremors, tachycardia, cardiac arrhythmias, seizures, polyuria/polydipsia, hyperthermia, cyanosis, and coma. Histologic lesions in the brain or spinal cord are usually lacking. The exact toxicological mechanism of action of caffeine is not known but may include inhibition of phosphodiesterase, enhanced catecholamine release, adenosine antagonism, or increased calcium entry into the cell. Caffeine toxicosis in animals most commonly occurs following the ingestion of chocolate; however, accidental poisonings may also occur from the ingestion of caffeine-containing products. Poisoning in a parrot resulting from chocolate ingestion has also been reported. Caffeine is well absorbed from the GI tract, and is highly metabolized by the liver to its inactive metabolites methyluric acid and methylxanthine. The plasma half-life of caffeine in dogs is approximately 4.5 hrs. The treatment of caffeine toxicosis in animals is usually symptomatic and supportive. Fluid therapy to enhance diuresis, anticonvulsants, antiarrhythmics, and repeated oral activated charcoal administrations are generally recommended. The oral LD50 of caffeine in the dog is approximately 140 mg/kg. Brunfelsia australis (yesterday, today, and tomorrow tree) This tropical tree is increasingly grown in the United States because of its colorful flowers and drought resistance. The flowers turn from purple (yesterday), to lavender (today), and white (tomorrow) as they mature. The smooth muscle relaxant scopoletin and the presumed convulsants hopeanine and brunfelsamidine have been isolated from this plant. Clinical signs reported in dogs with suspected Brunfelsia poisoning included vomiting, diarrhea, salivation, lethargy, moderate ptyalism, mydriasis, abdominal pain on palpation, bradycardia, “manic-type” behavior, ataxia, muscle fasciculations, rigidity (“sawhorse stance”), and seizures. Clinical management includes GI decontamination and symptomatic and supportive care including anticonvulsants. Lead The incidence of lead toxicosis in companion animals appears to be decreasing; nevertheless, it remains an important clinical problem. Historical sources of lead include lead-based paints, battery plates, certain caulking compounds and putties, linoleum, plumbing solder, roofing material, and asphalt. Water from lead plumbing, glazed crockery pots, and streams polluted by lead-contaminated effluent also may contain toxic concentrations of lead. Ingestion of older (pre-1978) lead-based household paints remains an important source of companion animal poisoning. Inhalation of lead may also pose a concern in confined areas where lead-based dusts or fumes are present (e.g. gun firing ranges). Generally, only 5–10% of ingested lead is absorbed in adults, but 40–50% is absorbed from the GI tract in juveniles, thus young animals are more susceptible to lead poisoning than are adults. Lead may dissolve to an appreciable degree in the acid environment of the stomach, greatly increasing its absorption. In contrast, metallic lead shots or bullets lodged in tissue do not readily dissolve except in joints or abscesses. Once absorbed, lead readily passes membrane barriers such as the blood–brain barrier and the placenta. Distribution is primarily to the kidney cortex, liver, and bone; the bone contains up to 98% of the total body lead burden. When the bone becomes saturated, signs of toxicosis may develop suddenly. Signs of lead toxicosis are generally referable to the nervous and GI systems. Neurologic signs with acute onset tend to predominate with higher exposure levels, while GI signs and chronic illness may be more common with lower exposures. However, both systems may be affected concurrently. In decreasing order of frequency, signs in dogs and cats are vomiting, seizures, anorexia, hysteria, and weight loss. In both dogs and cats, seizures are a common neurologic sign; therefore, lead toxicosis should be considered in any animal exhibiting seizures. EEG changes in dogs with lead-induced neurologic signs are not pathognomonic and may include intermittent or continuous high-amplitude slow wave activity. Hysteria is also commonly reported and is characterized by barking and crying continuously, running in many directions without purpose, indiscriminate biting of animate and inanimate objects, and other behavioral changes. Other neurologic signs include ataxia, tremors, and blindness. Animals may also develop aggression, dementia, pica, megaesophagus, and coma. Additional neurologic signs reported in cats include CNS depression, cerebellar or vestibular ataxia, and vertical nystagmus. Megaesophagus attributable to lead toxicosis has also been reported in cats. Differential diagnoses based upon history and clinical signs include rabies, canine distemper, epilepsy, encephalitis, spinal cord trauma, and other poisons. Lead poisoning likely remains more common in dogs than in cats. Blood-lead determination remains the single best indicator of relatively recent exposure to lead. Whole blood (heparinized or EDTA-containing tubes) rather than serum should be used since > 90% of circulating lead is bound to erythrocytes. Although a valuable indicator of exposure, blood-lead concentrations do not reflect the length of exposure, total body burden amount of lead, or severity of clinical signs. Other diagnostic laboratory tests include urine lead or urine δ-aminolevulinic acid (ALA) concentrations and blood zinc protoporphyrin (ZPP) levels. Signs of lead toxicosis may resolve fairly quickly following the initiation of therapy, despite concurrent high blood-lead concentrations. Cats may exhibit signs of lead toxicosis at lower blood-lead concentrations than dogs. The finding of numerous erythrocytes with basophilic stippling and/or immature (especially nucleated) erythrocytes without evidence of anemia is suggestive of lead poisoning; however, this hematologic effect is often lacking in dogs and cats. Other causes of nucleated red blood cells (e.g. endotoxemia, leukemia, splenic diseases) should also be considered. Paint chips or other lead objects within the GI tract may occasionally be detected on abdominal radiographs. Radiopaque material in the GI tract helps to rule in lead, but negative findings do not rule it out. For postmortem diagnosis, fresh frozen liver and kidney tissue should be submitted for lead chemical assay. Histologic changes potentially induced by lead include renal tubular necrosis and amorphous acid-fast intranuclear inclusions in hepatocytes and renal tubular epithelial cells. Lesions occur in the CNS (lead encephalopathy) as well as in the PNS (lead neuropathy). The likely primary lesion in acute human lead encephalopathy is breakdown of the blood–brain barrier, and similar effects are also observed in animals. Brain capillaries may be dilated, narrowed, necrotic, or thrombosed, and endothelial cells often swell. The consequent extravasation of fluid results in cerebral and cerebellar edema. Accompanying these vascular changes is neuronal necrosis (cerebrocortical and Purkinje cells) with secondary reactive gliosis and astrocytic scar formation. Neuronal lesions may be caused directly by lead rather than by defective vascular function since neuronal necrosis without vascular injury has been observed in acute experimental lead toxicity. In the PNS, lead neuropathy in humans, experimental animals, and cats is manifested by Wallerian axonal degeneration and segmental demyelination affecting primarily motor nerves. The treatment of lead poisoning is directed at controlling seizures and other life-threatening signs, removing lead from the GI tract, elimination of absorbed lead from soft tissue and bone, and identifying the source in order to prevent further exposure. Small pieces of metallic lead and lead paint chips can be removed from the GI tract with emetics and saline cathartics. Larger lead objects in the stomach or intestine may require endoscopic or surgical removal. Historically, the most commonly used chelator for lead has been CaNa2EDTA. This agent has been used in a variety of animal species. In both cats and dogs, CaNa2EDTA is given subcutaneously at 18.75 to 27.5 mg/kg, every 6 hrs, for 2–5 days. Prior to injection, CaNa2EDTA should be diluted to a final concentration of 10 mg/mL in 5% dextrose solution. Higher concentrations of CaNa2EDTA may cause pain at the injection site. Intravenous injection may be more effective, but requires additional labor resources. Continuous CaNa2EDTA therapy should not last more than 5 days because of CaNa2EDTA’s effects on the normal growth of the intestinal epithelium, enhanced zinc elimination, and nephrotoxicity. However, multiple treatments are occasionally necessary and each 5 days of treatment should be followed by a 5-day rest period. The clinical condition of the animal and blood-lead measurements is used to determine when to stop therapy. Due to CaNa2EDTA-induced increases in zinc elimination, oral zinc supplementation at 2 mg/kg may be necessary in dogs on low-zinc diets or those given multiple chelation treatments. D-penicillamine has also been recommended for asymptomatic dogs as a home-based follow-up therapy to inpatient chelation therapy, especially in animals with persistently elevated blood-lead concentrations. D-penicillamine is given orally at 110 mg/kg daily for 2 wks followed by a 1-wk rest. To prevent chelation of essential metals in the diet, D-penicillamine should be administered on an empty stomach (30 min before feeding). It may be necessary to divide the daily dose and give it every 6–8 hrs or decrease the total dose to 33–55 mg/kg daily if adverse effects—such as vomiting, listlessness, or anorexia—persist. Antiemetic drugs may also be administered 30 min to 1 hr before the D-penicillamine to reduce vomiting. D-penicillamine is well-absorbed from the GI tract. D-penicillamine is metabolized by the liver with very little excreted unchanged, and elimination is primarily via the kidney and liver. Side effects associated with D-penicillamine use include reversible proteinuria and hematuria. It may also enhance the absorption of lead from the GI tract; therefore, GI tract decontamination prior to chelation therapy and prevention of further exposure to lead are important. The use of 2,3-dimercaptosuccinic acid (DMSA) has dramatically improved lead chelation therapy. In contrast to other chelators, DMSA is a relatively selective, orally active, water-soluble chelating agent. Orally administered DMSA apparently does not increase lead absorption from the GI tract, and potentially may reduce it. It is still imperative to prevent ongoing oral exposure to lead; however, if re-exposure should occur (as in an outpatient setting), concurrent treatment with DMSA is unlikely to pose a particular risk. Side effects of DMSA reported in humans are limited. Elevations in serum alanine transaminase have been reported in humans, but these also may be induced by lead toxicosis. DMSA appears not to have a clinically significant effect on the excretion of essential minerals including calcium, magnesium, iron, copper, and zinc. Dogs and cats treated with DMSA (10 mg/kg of body weight, PO, q 8 hrs) for 10 days had reduced blood-lead concentrations and eliminated clinical signs of lead poisoning. Thiamine hydrochloride (2 mg/kg every 6 hrs) may be beneficial in lead-poisoned dogs, although its efficacy has not been proven experimentally. Another factor that should be considered is the possibility that children or adults may also have been exposed to lead from the same source as their pets. This is especially true if the exposure history includes possible risk factors for exposure like a recent remodeling of an older home that contains lead-based paints. Permethrin and other pyrethrin and pyrethroid insecticides Pyrethrins are natural insecticides, while pyrethroids (e.g. permethrin, fenvalerate) are more stable synthetic insecticides. Toxicological mechanisms of action of these insecticides include interference with sodium channels, enhanced sodium ion conductance, and postsynaptic γ–aminobutyric acid (GABA) receptor-chloride ionophore complex blockade. Pyrethrins and pyrethroids possess low acute oral toxicity to mammals (acute oral LD50s range from 25 to 10,000 mg/kg) due to their rapid hydrolysis in the GI tract and liver metabolism. Synergists (e.g. piperonyl butoxide, N-octyl-bicycloheptene dicarboximide [MGK 264]) are often added to insecticide formulations to decrease pyrethrin and pyrethroid metabolism and increase their insecticidal activity. It is likely that esterases involved in pyrethrin and pyrethroid insecticide metabolism may also be inhibited by prior organophosphorus or carbamate insecticide exposure, increasing the likelihood of pyrethrin toxicosis. The use of permethrin-based “spot-on” formulations (contain 45–65% permethrin) has been associated with toxicity in cats and occasionally dogs. Toxicosis generally develops within hours of exposure, but may be delayed as a result of prolonged exposure from dermal absorption or grooming. Initial clinical signs associated with the application of permethrin spot-on products include paresthesia manifested by ear twitching, paw and tail flicking, hyperexcitability, and hyperesthesia. Paresthesia to spot-on products may be treated by applying a vitamin E-based oil, olive oil, or corn oil on the site of insecticide application. More severe clinical signs associated with the development of pyrethrin or pyrethroid insecticide poisoning in cats and dogs include muscle tremors, increased salivation, ataxia, vomiting, CNS depression, hyperexcitability or hyperactivity, seizures, dyspnea and death. The toxic syndrome is considered reversible in most sublethally exposed animals with complete recovery often occurring within 72 hrs. Gross or microscopic lesions are typically absent. Chemical analysis for insecticide residues on the skin or in the GI tract of exposed animals may be used to confirm topical or oral exposure to these agents. Elevated tissue concentrations of these insecticides, especially brain and fat, may also help to support a tentative diagnosis of lethal poisoning. Direct correlation between tissue concentrations and severity of clinical signs (including death) for most insecticides has not been determined for cats or dogs. Treatment of toxicosis is largely supportive (washing skin, GI tract decontamination, seizure control, fluids, oxygen, and respiration support). Atropine (0.04 mg/kg, SQ) may diminish the degree of salivation and diarrhea in pyrethrin- or pyrethroid-poisoned cats. Unlike its use in organophosphorus or carbamate insecticide toxicosis, however, atropine is not considered a direct antidotal therapy for pyrethrin or pyrethroid insecticide poisoning. Methocarbamol (50 mg/kg, IV, repeat as needed to a maximum dose of 330 mg/kg/day) has been advocated for the control of permethrin-induced muscle tremors. ILE therapy has also been advocated for resolving cases in which animals have severe CNS signs (e.g. severe tremors, seizures). Ataxia, paresis, and paralysis are gait alterations associated with dysfunction of the central or peripheral nervous systems. Ataxia is defined as a failure of muscle coordination, paresis as a partial loss or impairment of motor function, and paralysis as complete loss or impairment of motor function in a body part. The reader is referred to Box 23.2’s potential toxic agents Some of these agents may also result in respiratory paralysis (e.g. aminoglycoside antibiotics). Cycad palms Cycads are a relatively primitive subtropical and tropical seed plant with a stout woody trunk and large stiff evergreen leaves. The starch obtained from the stems and processed seeds from certain cycad species is used as food by some indigenous tribes. An interesting association between the incidence of an amyotrophic lateral sclerosis/Parkinson’s disease complex (ALS/PDC) and the consumption of cycad-based food products among certain inhabitants of the island of Guam has been of broad interest to the neurotoxicology community. The cycad neurotoxin β-methylamino-L-alanine (BMAA) has been postulated to play a role in these diseases. Experimental studies performed in rhesus monkeys has shown that BMAA ingestion can result in limb muscle atrophy, nonreactive degeneration of anterior horn neurons, degeneration and partial loss of pyramidal neurons of the motor cortex, and conduction deficits in the central motor pathway. It appears that BMAA may induce its neurotoxic effects via an excitatory toxic mode of action. Excitotoxic cell death involves prolonged depolarization of neurons, changes in intracellular calcium concentrations, and the activation of enzymatic and nuclear mechanisms of cell death. The main excitatory amino acid receptors are quisqualate/α-amino-3-hydroxy-5-methyl-4-isoxazolepropionic acid (AMPA), N-methyl-d-aspartate (NMDA), and metabolic glutamate receptors (mGluR), all of which are activated by glutamate and similar substances. This mode of action is shared by many other neurotoxicants. Cycad poisoning in species of veterinary relevance also occurs. Cattle can be affected with neurologic and hepatic-gastrointestinal diseases appearing as somewhat distinct toxic syndromes. Neurologic manifestations include ataxia, lameness, conscious proprioceptive deficits, and axonal degeneration in the spinal cord and degeneration of the spinal ganglia. The most common signs in dogs ingesting cycad plants are associated with the GI and hepatic systems and include vomiting (± blood), diarrhea (± blood), and anorexia. Severe GI signs and hepatic damage with icterus and markedly elevated bilirubin concentrations and serum alanine aminotransferase and alkaline phosphatase activities often develop rapidly (within 24 hrs of consumption). These clinical signs are most likely due to the presence of the toxin methylazoxymethanol in the cycad plant. Neurologic signs occur in approximately 50% of all cases and include CNS depression, proprioceptive defects, coma, and seizures. A paralytic syndrome similar to that seen in people and cattle has not to date been reported and may reflect the need for repeated exposure to the cycad neurotoxin. A similar chronic paralytic effect (neurolathyrism) is seen in people and livestock that consume grass pea (Lathyrus sativus). Neurolathyrism is likely associated with the consumption of the glutamate structural analogue oxalyldiaminopropionic acid (ODAP). Treatment of cycad toxicosis primarily depends upon supportive care (e.g. GI tract decontamination, seizure control, fluids, oxygen, respiration support). Moxidectin Moxidectin is a second-generation macrocyclic lactone used for parasite control in animals. The principal mode of action of moxidectin is binding to γ-aminobutyric (GABA) and glutamate-gated chloride channels. Numerous preparations of moxidectin are available, including tablet and sustained release injectable for the prevention of heartworm disease in dogs, injectable and oral drenches for sheep, a pour-on formulation for cattle and deer, and sustained release injectable formulations for cattle and sheep. Unlike certain other macrocyclic lactones (e.g. ivermectin), moxidectin is poorly transported by p-glycoproteins. In general, moxidectin is slowly absorbed from the GI tract, and fecal excretion of the parent compound is the main elimination pathway. Clinical signs reported in dogs poisoned with moxidectin include an acute onset of seizures followed by paralysis and coma. Other clinical signs have included ataxia, generalized muscle tremors, paresis, hypersalivation, temporary blindness, and disorientation. Toxic doses associated with these neurologic effects ranged from 1.89 to 2.85 mg/kg. Moxidectin toxicosis can be managed initially using GI decontamination with supportive care (e.g. diazepam, glycopyrrolate, IV fluids, and mechanical ventilation) as needed. Moxidectin toxicosis has also been treated using ILE therapy. In one canine case, an emulsion of 20% soybean oil in water was administered intravenously as a bolus of 2 mL/kg followed by 4 mL/kg/hr for 4 hrs beginning 10 hrs after exposure and was administered again at a rate of 0.5 mL/kg/min for 30 min beginning 25.5 hrs post exposure. Mild improvement was seen after the first dose, and dramatic improvement was noted within 30 min of the second dose. The puppy’s neurologic status returned to normal within 6 hrs of the second administration, with no relapses. Box 23.3 lists poisons associated with CNS depression. CNS depression, stupor, and coma all represent states of altered consciousness. “Depression” connotes a lethargic animal that is incapable of responding to the environment in a normal manner.
Neurotoxicological Syndromes
Introduction45
Diagnostic considerations8, 16, 19, 74, 88, 89, 108, 146, 147, 158
Treatment considerations36, 40, 61, 66, 68, 70, 73, 120, 144, 195
Poisons associated with CNS stimulation and seizures6, 9, 11, 15, 21, 22, 33, 37, 41, 44, 47, 56, 60, 63, 67, 69, 71, 76, 79, 84, 90, 97, 99–101, 109–111, 113, 119, 123, 126–128, 130, 135, 139, 152, 168, 170, 171, 181, 185, 187, 189, 191, 192, 200
Toxicants causing paralysis1, 34, 38, 40, 46, 78, 132, 154, 161, 169, 197, 198
Poisons associated with CNS depression, stupor, or coma10, 12, 23, 31, 59, 81, 85, 87, 91, 93, 104, 105, 117, 118, 121, 140, 148, 150, 151, 157, 178, 179, 188, 193
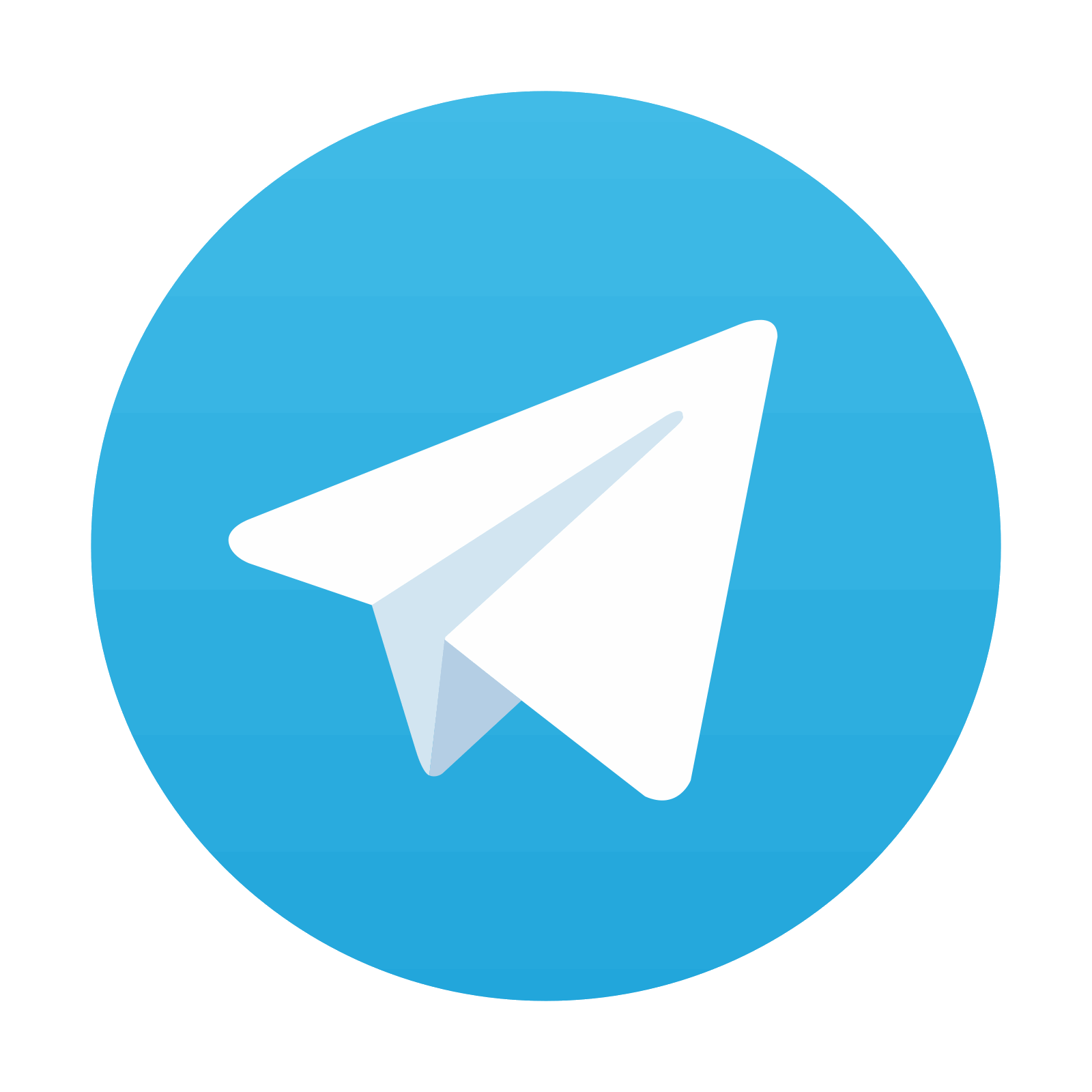
Stay updated, free articles. Join our Telegram channel
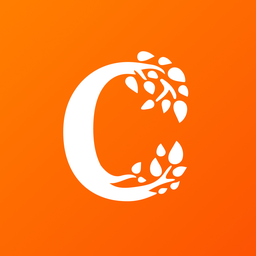
Full access? Get Clinical Tree
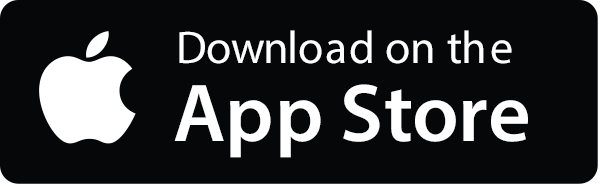
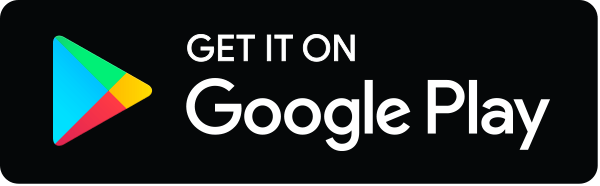