Rajendra Kumari, Davy Ouyang, and Henry Li Crown Bioscience Inc., 16550 W. Bernardo Dr. Building 5, San Diego, CA, 92127, USA Interrogation of the immune cell–tumor interactions has largely been dependent on immune competent mouse models in which cross reactive or surrogate immuno‐oncology (IO) agents can be tested to understand mechanism of action (MOA) and predict efficacy. These models include the transplantation of mouse cell lines into syngeneic immune‐competent hosts or genetically engineered mouse models (GEMMs), with their respective pros and cons (Talmadge et al. 2007; Li et al. 2017). Syngeneic models are simple and rapid to establish, but the repertoire of mouse tumor lines is relatively small compared to the diverse collection of human cell lines and many were established from tumors initiated by chemical mutagenesis, are maintained in vitro for many generations and lack resemblance to the human genetic disease. GEMMs in contrast provide a better model of human cancer with genetic features observed in human disease, such as specific oncogenes or tumor suppressors, replicated in a mouse through engineering (transgene, knock‐in, or knockout). A wide range of well‐characterized models, with defined molecular pathogenesis, have been made available in the past few decades. However, the major limitation of GEMMs is that development and validation of these models is time‐consuming, laborious, variable, expensive, and therefore inadequate for pharmacology evaluation. Novel and robust immunocompetent mouse tumor models are needed to overcome issues commonly associated with syngeneic tumor models and GEMMs. Homograft of spontaneous/autochthonous tumors could be alternative models of choice for IO drug evaluation by overcoming some of the shortfalls of the above two models. The spontaneous/autochthonous tumor fragments are implanted into mice of the same background strain, creating a system combining the strengths of GEMM with improved operational simplicity, consistency, and growth of transplanted tumors. This chapter describes different tumor homograft models, their utility in “preclinical and clinical trials” and how they compare with standard models. In the last few years, syngeneic models (also referred to as mouse homograft tumors), have widely been used in preclinical studies as the preferred transplanted tumor platform (as opposed to human xenograft tumors). This is due to the increase in IO research and for these models being the only viable system which can be easily setup in immunocompetent hosts to investigate MOA and efficacy of cross reactive or surrogate immunotherapeutics before moving to complex human studies (Li et al. 2017; Gould et al. 2015; Dranoff 2012). These immunocompetent models utilize in vitro immortalized mouse cancer cell lines, developed from either spontaneously arising tumors in older mice or from carcinogen induction. The syngeneic cell lines are readily accessible from commercial tumor cell banks with limited licensing restrictions. Typically, these tumors are rapid to establish by simple engraftment, frequently subcutaneously (SC), into the same inbred mice. The synchronized transplantation enables robust pharmacology evaluation involving large numbers of tumor models. These cells lines can also be engineered to express reporter such as luciferase, allowing orthotopic, or metastatic modeling for real time, longitudinal monitoring, and synchronization for pharmacology studies (McKenzie et al. 2017). Further, there is a wealth of published information about these models, making them the “default industry standard.” While transplanting cell lines derived from established tumors does not precisely replicate the interplay and coevolution between the tumor and its microenvironment (TME), it does allow investigators to test novel immunotherapies that activate immunity or inhibit immuno‐suppression of antitumor immunity. Most checkpoint inhibitors (CPI) were first confirmed in syngeneic models as proof of concept (POC), e.g. anti‐PD‐1 and anti‐CTLA‐4 antibodies with MC38 tumors (Selby et al. 2016). Now, many syngeneic models (>20 known) have been extensively profiled genomically, immunologically, and pharmacologically for IO agent efficacy by various laboratories (Mosely et al. 2017; Yu et al. 2018; Lechner et al. 2013), including ours (Jin et al. 2018, 2019). Immune profiling, or immunophenotyping, of syngeneic tumors usually involves the determination of tumor‐infiltrating leukocytes (TILs) of different lineages, e.g. lymphocytes (T‐ and B‐cell), macrophages (TAMs), natural killer cells (NKs), and tumor‐resident dendritic cells (DCs), the relative percentage within tumors, as well as their activation status and their location. Flow cytometry is commonly used to determine the numbers and relative percentage of different subsets of immune cells in tumors, spleen, lymph nodes, and peripheral blood (Yu et al. 2018; Mosely et al. 2017; Lechner et al. 2013), while immunohistochemistry (IHC) is commonly used to determine their locations. The assessment can be carried out at baseline (pretreatment, as predictive factors) and for pharmacodynamic (PD) effect in response to CPIs. Immunophenotyping, along with immune‐genomic profiling, at baseline and/or as PD effect, along with efficacy data associated with surrogate CPI treatment, have been often benchmarked to facilitate model selection (https://mubase.crownbio.com). Syngeneic models have become, and probably stay, the work horse for drug industry IO in vivo studies for some time to come. However, there are several limitations for using syngeneic models; Unlike transplanted syngeneic cell line models, autochthonous models arise spontaneously, thereby creating favorable microenvironments for tumor progression through the manipulation of various oncogenic pathways in different tumor types and inducing immune editing and immune tolerance. Autochthonous models include GEMMs as well as chemical, viral, or physical carcinogen‐induced tumors (Talmadge et al. 2007), which will be briefly discussed to highlight their relevance to immunotherapy and the rationale for developing tumor homografts. GEMMs have made a significant contribution to cancer research in the last 30 years and increased our understanding of the molecular pathways responsible for initiation and progression of human cancer and highlighted the importance of specific oncogenes and tumor suppressor genes (TSG) in particular types of cancer. One or several genes putatively involved in malignant transformation are deleted, mutated, or overexpressed to recapitulate genetic/molecular changes in human cancers. As a result, GEMM tumors driven by loss‐of‐function (LOF) of TSG and/or gain‐of‐function (GOF) of oncogenes, spontaneously arise and progress in mice (Day et al. 2015; Talmadge et al. 2007) accompanied by profound immune suppression and escape from immune surveillance, recapitulating key features of de novo human tumorigenesis. This is in contrast to transplanted syngeneic models which involve engraftment of fully progressed tumors into a naïve host, inevitably resulting in an acute immune response that may not be relevant to the natural course of disease. GEMMs therefore provide a more physiologically relevant TME that is ideal for IO research and drug testing for accurate prediction of clinical response. The spontaneous tumorigenesis in GEMMs, reflective of natural human tumorigenesis, enables modelling of both preventive and therapeutic interventions, including adjuvant or neoadjuvant treatments (Liu et al. 2016), prophylactic treatment or preventative vaccines, etc., to guide clinical testing. There is a large collection of GEMM available covering many common cancer indications, such as pancreatic, lung, prostate, breast, colon, etc. They have certain human tumor characteristics of histopathology, TME (Pitt et al. 2016; Smyth et al. 2016), stem‐cell, and differentiations in contrast to the limited syngeneic cell line collection. They can usually be accessed through banks, academic institutions or generated in‐house. The technology for deriving GEMMs has also evolved over the last 30 years. Traditional GEMMs were created by introducing germline mutations, through transgenes or targeted knockout (KO)/knock‐in (KI), which resulted in genetic alterations in all of the cells of the animal which has been criticized as it certainly does not reflect the patient. The development of conditional or non‐germline GEMMs provided more sophisticated models to control tumor onset and progression in an inducible, and cell lineage‐/tissue‐specific manner. More recent technology advancements in gene editing (i.e. CRISPR‐Cas9), embryonic stem (ES) cell culture, and viral delivery, have generated GEMMs with on‐and‐off, or more precise disruption of oncogenes/tumor suppressors in the targeted tissues/cells in animals, more closely mimicking what occurs in human cancers. What is even more attractive is that the timeline required for generating these non‐germline GEMMs is significantly shorter than traditional GEMMs or avoids embryonic lethality. Various types of GEMMs have been reviewed elsewhere (Livshits and Lowe 2013; Day et al. 2015; Heyer et al. 2010), representing a variety of histiocytic cancer types driven by multiple independent drivers. Many GEMMs with specific druggable disease pathways intentionally engineered to mimic specific cancers have been intensively studied and targeted pharmaceutically. They are particularly equipped for testing targeted and IO therapies, or the combination of both, in comparison to conventional syngeneic models which are not driven by clinically relevant pathways (Mosely et al. 2017). EGFR and KRAS mutants are two of the most prevalent oncogenic drivers in human lung adenocarcinomas patients, which leads to histopathologically indistinguishable, yet molecularly distinct tumors in mice. These tumors respond to treatments such as EGFR inhibitors and CPIs, very differently. GEMMs with mutant EGFR significantly induce PD‐L1 expression on tumor cells, leading to checkpoint blockade of T‐cells (Akbay et al. 2013). These tumors also produce cytokines e.g. IL‐6 and progranulin, and recruit regulatory T‐cells (Treg) and suppressive macrophages to induce further immune suppression. They have been shown to respond favorably to anti‐PD‐1 treatment. However, GEMMs with mutant KRAS driven tumors usually have low PD‐L1 levels and are insensitive to anti‐PD‐1 antibodies. Alternatively, in a lung squamous cell carcinoma (SCC) GEMM model, PTEN and LKB1 loss is associated with PD‐L1 expression on the tumor cells and an enrichment of tumor‐associated neutrophils, a distinct mechanism of immune escape in contrast to adenocarcinoma (Xu et al. 2014). These findings have highlighted the value of GEMMs in interrogating the interaction between a tumor cell and TME, and understanding MOAs of antitumor immune responses. GEMMs therefore provide the most complete representation of cancer development of all the murine cancer models available. However, GEMMs are also the most challenging to work with for pharmacology studies including, but not limited to, the following constraints: In addition to GEMMs, the non‐engineered autochthonous tumors can be induced with the same utility as GEMMs, as compared to transplanted tumors, with spontaneous orthotopic growth, closely mimicking human tumor histology and metastasis via lymphatic and vascular vessels surrounding (Berger 1999). A study comparing the outcomes from a chemically induced autochthonous models in rats and GEMMs with a meta‐analysis of clinical outcomes (Corpet and Pierre 2005) found that carcinogen‐induced tumors correlated best with clinical responses. However, like GEMMs, these non‐engineered autochthonous tumor models have an inherent variability in tumor onset (time and frequency), and thus the number of animals required for a study, leading to less frequent use for drug development. Further, in a post‐genomic era, the non‐engineered autochthonous models have been replaced by GEMMs to an extent. Although no model can perfectly capture the human condition, several autochthonous models provide the most complete representation of cancer development, from initiation through to progression, coevolving with intrinsic stroma, and possessing an intact immune system thus providing the only opportunity to evaluate drug delivery, therapeutic response, and biomarker expression. In addition, these complex dynamic processes contribute to overall disease properties, and in particular, constitute a source of the inter‐ and intra‐tumoral heterogeneity that challenges successful therapeutic development and prevention therapies. In general, pharmacology modelling using autochthonous models is time‐consuming and requires “rolling enrolment,” in addition to cost, prohibiting wide adaptation for preclinical testing. This has prompted the urgent need of a more robust preclinical platform that can combine the strengths of GEMM and transplanted tumors. Homografts of spontaneous murine tumors from autochthonous sites, referred to as “mouse‐derived tumor homografts,” combine the genetic and pathological human cancer similarities of autochthonous models with the relative ease of transplantation of PDX and can be described as “the mouse version of PDX.” They prospectively therefore have a better translatability than conventional syngeneic cell line‐derived mouse tumors (Day et al. 2015), creating a robust preclinical platform that might industrialize pharmacology evaluation with improved operational simplicity. These mouse‐derived tumor homografts are typically established using tissue fragments of primary tumors in autochthonous models via subcutaneous (or orthotopic) transplantation into immunocompetent syngeneic hosts. Once the tumor has reached an appropriate size (e.g. 700–1000 mm3) it is then expanded through sequential passaging into multiple mice (Figure 3.1). Subsequently, they therefore have obvious advantages over autochthonous tumors in operational simplicity for pharmacology research, large cohort production, tumor growth synchronization, and higher throughput efficacy studies with short timeframes (three to eight weeks). Figure 3.1 Schematic showing the generation of mouse‐derived homografts. Devoid of in vitro growth in plastic, unlike standard syngeneic cell lines, these models also better recapitulate the original disease by keeping the characteristics of the primary cancer stem cell‐driven diseases, heterogeneous histo‐ and molecular pathology, and high genetic stability, without incurring irrelevant genetic shifts needed for in vitro growth. Like their PDX counterparts, these models are expected to have improved predictive power (Tentler et al. 2012). Like GEMMS, they also have complete mouse immuno‐competency, enabling interactions among tumor cells and their intrinsic microenvironments and are suitable for surrogate cancer IO, targeted agents as well as combination therapies. The sources of these homografts can be any spontaneous mouse tumors, including those from GEMMs and those due to aging or chemical carcinogens induction, thus increasing repertoire of cancer types and diversity of transplanted mouse tumor models, as shown in a summary of published models (Table 3.1). Table 3.1 Summary of published tumor homograft models. Like their PDX counterparts, homografts require maintenance and expansion in donor mice which is achieved through sequential passaging of tumor material. These tumor fragments are reported to better reproduce the histological nature of the primary tumor, with original 3D architecture and cell‐to‐cell contact, known to influence signaling, thus to potential drug efficacy. In addition, orthotopic implantation of fragments may increase metastasis efficiency compared to cell suspensions (Khanna and Hunter 2005; Hoffman 1999) and avoids artificial diffusion of injections of cell suspensions. However, implantation of tumor fragments with unknown cell number and regional heterogeneity within the tumor can lead to higher inter‐ and intra‐study variability. In a study using mammary tumors from multiple GEMM models into naive recipients, generating multiple small similar‐sized fragments was reported to limit the number of secondary recipients (Varticovski et al. 2007). Tumor banks can be generated with either fragment or cell suspensions depending on which method yields higher cell numbers for banking as well as a higher percentage of viable cells both pre‐freeze and post‐thaw. Pooling cells from several tumors, or expanding individual tumors in secondary recipients, can generate a virtually unlimited, on‐demand source of tumor cells for preclinical studies. In our experience of developing multiple different tumor homografts, the fragment‐based approach has been easily adopted across majority of models (Wang et al. 2019a; An et al. 2018), with the option of switching to cell suspensions on a case by case basis to determine if this improves the growth characteristics of the model. The following method describes the fragment‐based approach;
3
Mouse Tumor Homografts Recapitulate Human Molecular Pathogenesis
3.1 Introduction
3.2 Transplanted Syngeneic Cell Line Models
3.3 Spontaneous Autochthonous Mouse Models
3.4 Mouse‐Derived Tumor Homografts
Cancer type
Parental model
Strain
Homograft model reference
Breast
MMTV‐PyMT
FVB/N
Maglione et al. (2004), Varticovski et al. (2007)
Breast
K14cre; Brca1F/F; p53flox/flox
FVB/Ola
Rottenberg et al. (2008)
Breast
K14cre; Cdh1F/F ; Trp53flox/flox
FVB/Ola
Evers et al. (2010)
Bladder
Kras(G12D/+); Ptenflox/flox
C57Bl/6
Wang et al. (2019b)
Lung
K‐Raslox/LSLG12Vgeo or EML4ALK
NU‐Foxn1nu
Ambrogio et al. (2014)
PDAC
Pdx1‐Cre; Kras(G12D/+); Trp53−/− (KPC)
C57Bl/6
An et al. (2018), Tu et al. (2018)
Prostate
Pbsn‐Cre; Kras(G12D/+); Ptenflox/flox
C57Bl/6
Wang et al. (2019a)
Sarcoma
Trp53−/−
C57Bl
Wang et al. (2019a)
Skin SCC
APCmin/+
C57Bl/6
Wang et al. (2019b)
3.5 Generation of Tumor Homografts
Protocol
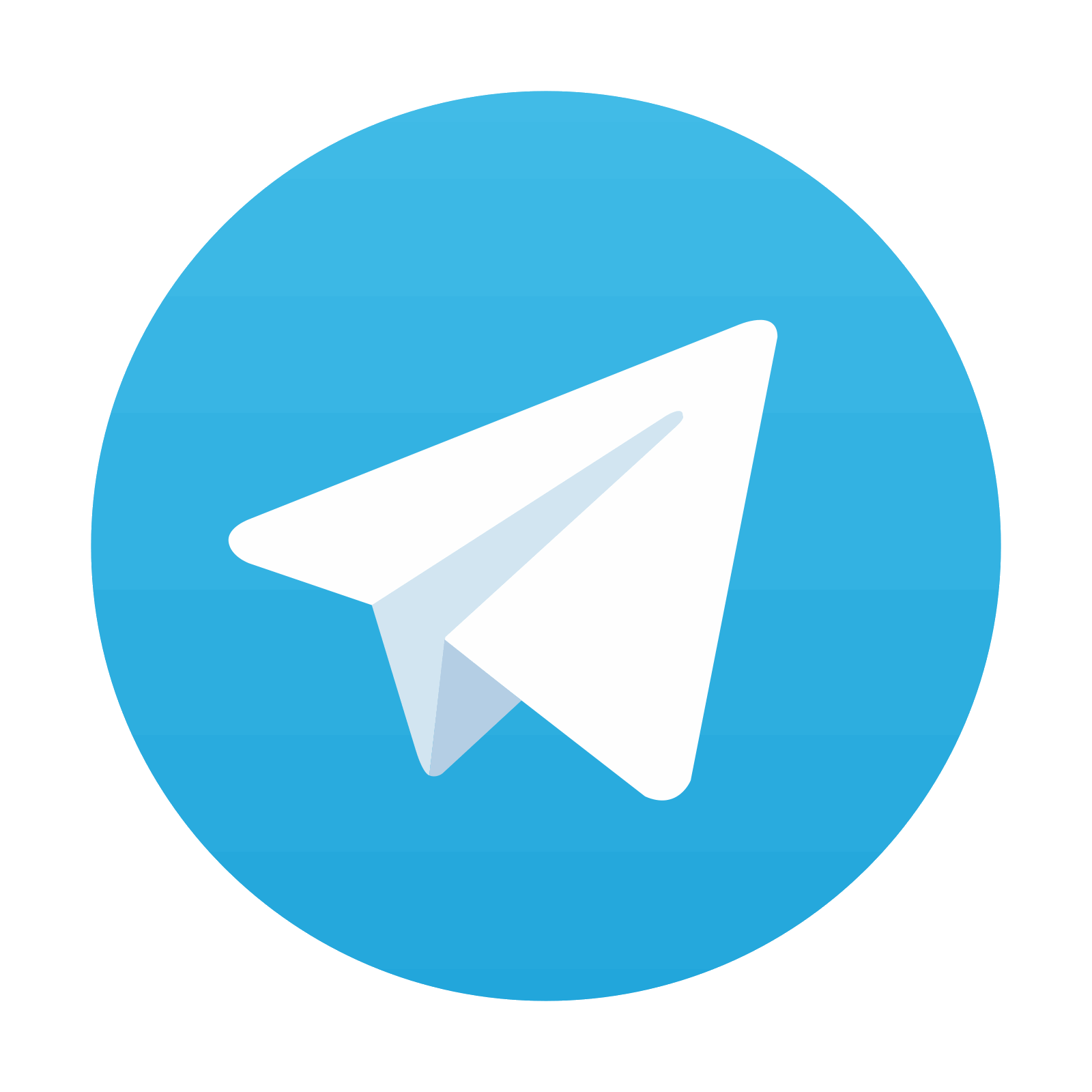
Stay updated, free articles. Join our Telegram channel
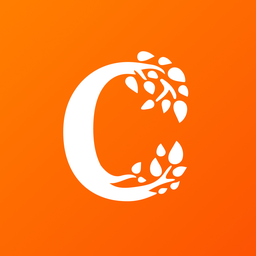
Full access? Get Clinical Tree
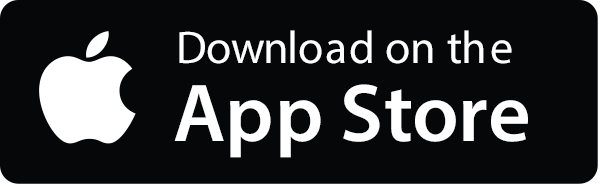
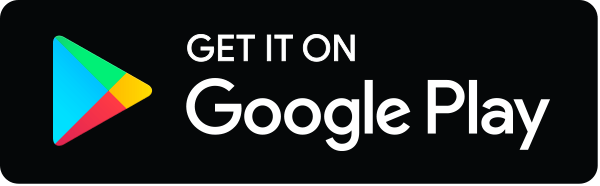