The ISAG Panel defines the minimum number of markers to be used for horse identification and parentage analysis. In practice, panels in current usage contain 17–20 markers. Such panels have a very high level of performance for individual identification and parentage testing, based on conventional metrics that assume random mating. Because horse-breeding practices often involve mating of close relatives, information from additional markers may be needed for specific situations. By recommendation of the Horse Standing Committee in 2010, a set of 13 STRs (TKY287, TKY294, TKY297, TKY301, TKY312, TKY325, TKY333, TKY337, TKY341, TKY343, TKY344, TKY374, and TKY394) from the panel developed by Tozaki et al. (2001) has been designated as the secondary panel. Measures of genetic variability and exclusion probabilities for the two panels obtained from records of the VGL (available at http://www.vgl.ucdavis.edu/genomic/Supplemental%20Table%2018.S1.pdf) demonstrate the usefulness of the two panels across 26 breeds.
Horse racing and forensic analysis
Horse identification and sample matching is becoming a more common practice in the horse racing industry. Proper identification of horses competing in official races and maintenance of legal chain of custody of samples undergoing drug testing are important in doping control regulations. Positive results from drug tests can be challenged by claims of sample switching. The STR panels described above provide the necessary level of discrimination to match DNA profiles of a horse obtained from blood or hair root with those obtained from urine samples submitted to a drug-testing laboratory. A multiplex panel with 24 STRs with 21 horse and 3 human markers has also been developed and validated for Thoroughbreds and Standardbreds to address the specific requirements of doping control in racetracks, including detection of fraudulent contamination of horse samples with human urine (Chen et al., 2010). Horse genotyping laboratories are usually equipped to handle forensic cases.
Genetic Tests for Genetic Diseases
Since the molecular basis of a horse genetic disease was first described (hyperkalemic periodic paralysis or HYPP) (Rudolph et al., 1992), there has been a steady stream of discoveries of the causative mutations for other inherited defects. DNA tests offered by commercial laboratories are currently available for 10 genetic diseases – glycogen branching enzyme deficiency (GBED), hereditary equine regional dermal asthenia (HERDA), HYPP, lethal white overo foal syndrome (LWO), two forms of junctional epidermolysis bullosa (JEB), lavender foal syndrome (LFS), malignant hyperthermia (MH), polysaccharide storage myopathy type 1 (PSSM1), and severe combined immunodeficiency (SCID). Details about the specific mutations and horse breeds in which they occur are provided in Table 18.2. Reviews of the conditions and the supporting genomic research are covered in other chapters. None of the causative mutations are restricted to one breed. As reviewed by Finno, Spier, and Valberg (2009), genetic testing for each disease is applicable to related breeds and crossbred animals such as, for example, GBED, HERDA, and HYPP in Quarter Horses, Appaloosa, American Paint horse, and Quarter Horse crossbred animals; SCID in Arabians and part-Arabians; JEB (LAMC2) in Belgian, Breton, Comtois, Vlaams Paard, and Belgische Koudbloed Flander; and PSSM1, which has widespread occurrence among breeds.
Recent work by Bellone et al. (2010) identified TRPM1 (Transient Receptor Potential Cation Channel, Subfamily M, Member 1) as the gene responsible for the white spotting pattern known as leopard complex (LP), or Appaloosa spotting. Homozygosity for the LP allele is associated with the eye defect known as congenital stationary night blindness (CSNB). A non-coding SNP (ECA1:108249293C>T), has been found to be in complete association with LP and CSNB in Appaloosas and Knabstruppers, and can be used as proxy in a diagnostic assay for zygosity of LP and CSNB. The causative LP mutation has since been identified and will soon become available from service laboratories.
PCR methods for detection of normal (wild-type) and disease-causing alleles are described in references listed in Table 15.2 or elsewhere as indicated next for specific diseases. The methods are based on restriction fragment length polymorphism (RFLP) procedures for GBED (Wagner et al., 2006), HERDA, HYPP, LFS, MH, and PSSM1; fragment length differences for both types of JEB and for SCID (Bernoco & Bailey, 1998), or allele-specific amplification for LWO (Metallinos, Bowling, & Rine, 1998). Laboratories providing genetic disease testing are found in many countries (e.g., Australia, Brazil, Canada, France, Germany, Italy, South Africa, Sweden, The Netherlands, the United Kingdom, and the United States). The specific tests offered by each laboratory vary according to local demand or licensing restrictions for patent protected tests. GBED, HERDA, HYPP, JEB, LWO, and PSSM1 are patented in the United States; SCID is covered by international patent in the United States, Canada, and the United Kingdom. In the United States, LFS is available only from Cornell University’s Animal Health Diagnostic Center, PSSM1 from the University of Minnesota’s Veterinary Diagnostic Laboratory, and SCID from VetGen (Ann Arbor, MI).
Table 15.2 Genetic disease and allelic variants identified by DNA testing.
Genetic disease testing is not subject to oversight for quality control of service laboratories or for standardization of allele nomenclature. As the primary users of the tests are breeders, owners, and veterinarians, a uniform designation of normal and disease alleles facilitates the understanding of results reported by different test providers. In Table 15.2 we propose a standard nomenclature based on assigning the letter N to the normal allele and a letter symbol for the disease allele that is easily associated with each condition.
Mandatory testing for genetic diseases is not standard practice among registries. Exceptions to this are the registration regulations of the American Quarter Horse Association (AQHA) for HYPP and of the U.S. and Canada Belgian Draft registries for JEB. In 1996, AQHA instituted the requirement of HYPP testing and disclosure of status on registration certificates for all foals that descended from the stallion Impressive and that were born on or after January 1, 1998. The rule was amended in 2004 to require parentage verification and HYPP testing of all of Impressive’s descendants born on or after January 1, 2007, and to make ineligible for registration HYPP homozygous foals. JEB became a mandatory test for Belgian stallions coming into service after November 1, 2002, for mares inseminated with frozen semen or used as embryo donors, and for the resulting foals.
HYPP, JEB, and SCID have the longest history of genetic testing. A survey of 12 age cohorts (1998 to 2009) of Belgians and Quarter Horses tested at the VGL (Table 15.3; Penedo, personal communcation) shows no change in disease allele frequency for JEB and an increase for HYPP of disease incidence and allele frequency. Information about SCID testing from the Arabian Horse Association (http://www.arabianhorses.org/education/genetic/docs/10Genetic_SCID_2010.pdf) suggests a pattern similar to JEB.
Table 15.3 Survey of JEB and HYPP incidence and disease allele frequency in 12 year of birth cohorts of Belgians and Quarter Horses.
Although the estimates are not based on random sampling within each year, the data for Quarter Horses suggests continued human-driven positive selection for HYPP in the halter subpopulation (Naylor, 1994; Tryon et al., 2009). The situation with JEB and SCID, both fatal at an early age, shows that carriers remain reproductively active. If other diseases follow the same trend of JEB and SCID, the defective alleles will remain in the gene pool of breeds and testing for these mutations will be relevant for a long time.
Genetic Tests for Phenotypic Traits
Horse coat color phenotypes have been collected and propagated by breeders since the early days of domestication. Analyses of ancient horse DNA samples provided evidence for rapid accumulation of color variants in the fossil record of early domesticates dating 5,000 to 2,600 years before present (Ludwig et al., 2009). Most modern breeds display variation in coat color resulting from action of several genes, with rare exceptions in which a specific phenotype is fixed (e.g., all black Friesian, all flaxen Haflinger). Coat color has long been the object of interest to breeders, intent on producing animals with particular colors that enhance the aesthetic and economic value of animals.
Since the discovery of the first coat color mutation in horses (Marklund et al., 1996), advances in horse genomic resources have accelerated such that the genes and mutations for most major color variants have now been identified. The genomic research of coat color traits is reviewed in depth in Chapter 11. We provide here information about diagnostic tests available to the horse industry based on published work. These tests determine genotypes that help with coat color classification of animals and that allow breeders to devise mating schemes for consistent production of foals with desired phenotypes.
The known genes and causative mutations associated with coat color variants are summarized in Table 15.4. The allele nomenclature for Extension and Agouti is in common usage among laboratories. For the dilution and white-spotting genes, the allele designations follow the same scheme used with genetic disease alleles, with N indicating absence of the mutation that defines the color. The 11 Dominant White KIT variants, all presumed to be homozygous-lethal (Haase et al., 2007; Haase et al., 2009), have not found wide application in coat color testing yet because of their restricted occurrence to specific lineages within breeds, very recent origin in most cases, and rare incidence. However, testing for presence of the W4 mutation (Haase et al., 2007) is now one of the requirements for registration with the Camarillo White Horse Association in the United States, which represents a small historic breed founded by one white stallion. In breeds with overo white spotting pattern, testing of breeding stock for presence of the mutation associated with Lethal White Overo Foal Syndrome is common practice among owners to reduce the risk of producing homozygous foals that are afflicted with ileocolonic aganglionosis and die shortly after birth because of functional intestinal obstruction.
Table 15.4 Coat color genes and allelic variants identified by DNA testing.
As mentioned in the previous section, a non-coding SNP in TRPM1, associated with leopard complex spotting (Bellone et al., 2010) in Appaloosas and Knabstruppers, can be used as a genetic marker for the pattern in these breeds and should soon become available from service laboratories. Other coat color phenotypes (e.g., dun dilution, roan, splash white) are currently under investigation and diagnostic tests for the specific mutations should become available in the near future. Multi-locus allele frequencies of coat color variants in different breeds are rarely documented in the literature. Data for 11 breeds in the United States obtained from the VGL (Table 15.5; Penedo, personal communication) illustrate the widespread occurrence of the variants in Extension, Agouti, Cream, and Gray loci, and the more limited distribution of Champagne and Silver dilutions, and white-spotting patterns, such as Sabino-1, Tobiano, and Lethal White Overo. We note that frequencies for TO and O in Paints may be overestimated because of a bias in testing for homozygosity and presence of O in overo-patterned horses, respectively. In a random sampling of Paint horses, the allele frequency of LWO was estimated to be 0.11 (Tryon et al., 2009).
Table 15.5 Allele frequencies of coat color variants in 11 horse breeds: Andalusian (AN), Appaloosa (AP), Gypsy Cob (GC), Morgan Horse (MH), Miniature Horse (MI), Paint (PT), Quarter Horse (QH), Rocky Mountain Horse (RM), Tennessee Walking Horse (TW), and Thoroughbred (TB).
Genotyping methods for individual loci are described in each of the original papers, but protocols for simultaneous genotyping of multiple coat color loci have been proposed based on SNAPshotTM (Kakoi et al., 2009) and real-time PCR (Royo et al., 2008) techniques. Other SNP-genotyping technologies and instruments available from companies such as Applied Biosystems, Fluidigm, KBioscience, Illumina, and Sequenom lend themselves to simultaneous genotyping for all known color variants, with the possible exception of Gray for which accurate genotyping relies on long-range PCR to detect presence and dosage of the 4.6 Kb tandem duplication.
Future Developments in Molecular Genetic Testing
DNA testing for identification and parentage verification is an integral part of the process to record pedigrees with breed organizations. The need for this application will continue to exist well into the future. The number of diagnostic tests stands to increase exponentially as a result of ongoing research on simple and complex traits related to health, coat color, reproduction, and performance. The recent report of a SNP associated with race distance aptitude in Thoroughbreds (Hill et al., 2010) provides an example of future trends in applied genomics; markers predictive of “performance” will be in demand and become an important component of genetic testing. As most trait-related mutations are simple base variations, use of SNP-genotyping technologies allow implementation of more streamlined and cost-effective procedures for simultaneous screening of multiple traits. SNP panels for identification and parentage have begun to emerge (see Hirota et al., 2010). Data from the EquineSNP50 chip are being mined and will contribute to the selection of a suitable standard SNP panel. Combining SNPs for parentage and economic traits in a single test is a reality in other livestock species, namely cattle. Future development in horse molecular genetic testing is poised to follow a similar path.
Karyotyping Services Available for the Horse
The basic chromosome analysis
The aim of clinical karyotyping or chromosome analysis is to determine whether or not a horse has a normal set of chromosomes. The analysis is usually carried out on animals with various reproductive and developmental disorders to ascertain the possible cause of the abnormal phenotype. Additionally, cytogenetic tests are ordered by insurance companies, horse breeders, and owners to evaluate potential breeding stallions, broodmares, or the horses to be purchased.
The analysis includes counting the diploid chromosome number – which for the normal horse is 2n = 64 – arranging homologous chromosomes into pairs and assessing their morphology, and ordering chromosomes into a karyotype according to the international standard (ISCNH, 1997). The analysis also involves identification of the sex chromosomes to count their number, check morphology and determine whether or not the genetic sex corresponds to the phenotypic sex. Female horses should have two X chromosomes and male horses should have one X and one Y chromosome.
Chromosome preparations are typically obtained from peripheral blood lymphocyte culture (see Raudsepp & Chowdhary, 2008; Durkin, Raudsepp, & Chowdhary, 2010). In case of suspected chimerism or mosaicism, chromosomes are also analyzed from cultured skin fibroblasts. For lymphocyte culture, 5–10 mL of blood is aseptically collected from the jugular vein in heparinized vacutainers and allowed to settle. Lymphocytes are obtained from the buffy coat and are cultured for 72 hours in medium supplemented with fetal calf serum, pokeweed mitogen, and antibiotics. The cultures are harvested with colcemid using standard approaches. The cells are subjected to hypotonic treatment and four rounds of fixation. Finally, the fixed cell suspension is dropped on a clean wet glass microscope slide to burst the cells and obtain metaphase spreads.
For basic chromosome analysis, the preparations are routinely stained with Giemsa, which is usually sufficient for counting the chromosome number and arranging chromosomes roughly into a karyotype. To further verify the sex chromosomes, the preparations are stained by C-banding (Arrighi & Hsu, 1971). The X chromosome shows a characteristic dark C-band at Xq21, which corresponds to facultative heterochromatin, while the predominantly heterochromatic Y chromosome stains overall dark (see Chapter 5 for details). An example of clinical cytogenetic analysis results is shown in Figure 15.1.
Figure 15.1 Cytogenetic analysis results for a mare with 63,XO karyotype: (a) a Giemsa stained metaphase spread; (b) the same metaphase spread arranged into a karyotype; and (c) C-banded metaphase spread showing the location of the single X chromosome (arrow).

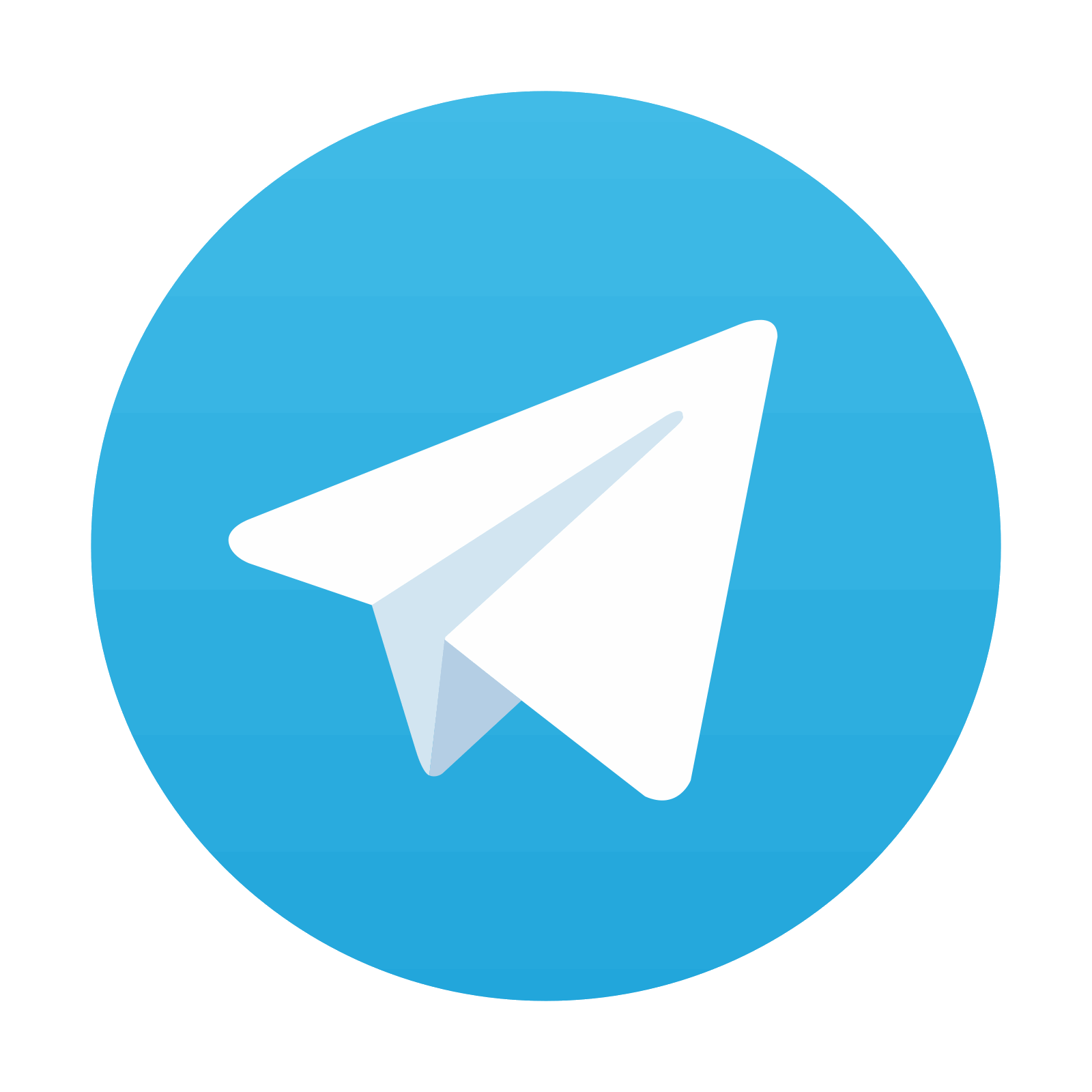
Stay updated, free articles. Join our Telegram channel
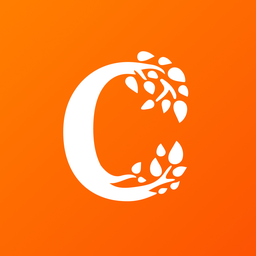
Full access? Get Clinical Tree
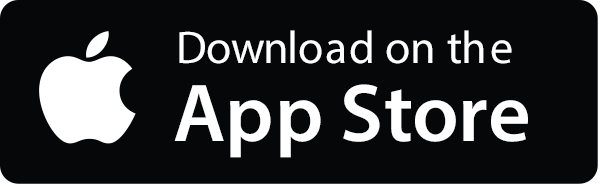
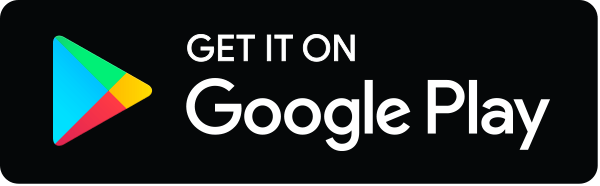