, Monika Hassel2 and Maura Grealy3
(1)
Centre of Organismal Studies, University of Heidelberg, Heidelberg, Germany
(2)
Spezielle Zoologie, Universität Marburg FB Biologie, Marburg, Germany
(3)
Pharmacology and Therapeutics, National University of Ireland Galway, Galway, Ireland
The ability to understand developmental processes requires appropriate organisms. The field of genetics established the precedent of focussing research on a few reference or “model” organisms such as Drosophila or maize. Recent developmental biology has depended on a small number of organisms for much of its spectacular progress. This concentration of effort facilitates attempts to advance in the analysis of the basic processes down to the molecular level. On the other hand, there is no single organism which could be selected to study all fundamental events and aspects of development; for each embryonic development leads to a single particular species, and not to an animal in general. From the egg of Drosophila a fruit fly arises, not an insect in general, not a fish and not a human being. General principles are only recognized when the events of development are studied in several diverse organisms, which develop differently yet display some common features. In addition, laboratory work quickly reveals that even the best model organism exhibits, in addition to its particular advantages, specific disadvantages. Thus the zebrafish has transparent embryos but large-scale genetic studies require hundreds of aquariums and a staff of workers, and the mouse provides a wealth of developmental mutants but cannot be conveniently frozen for long-term storage.
The main invertebrate model organisms that are currently used are shown in Fig. 4.1.
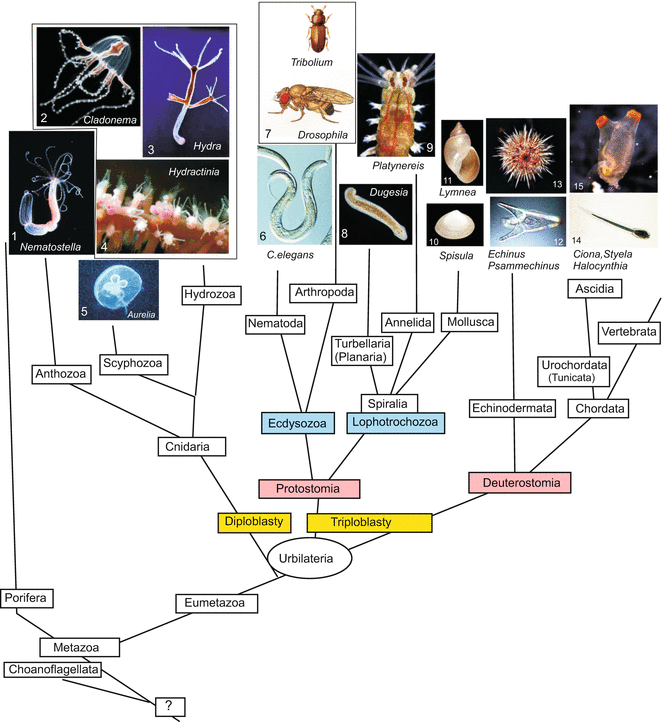
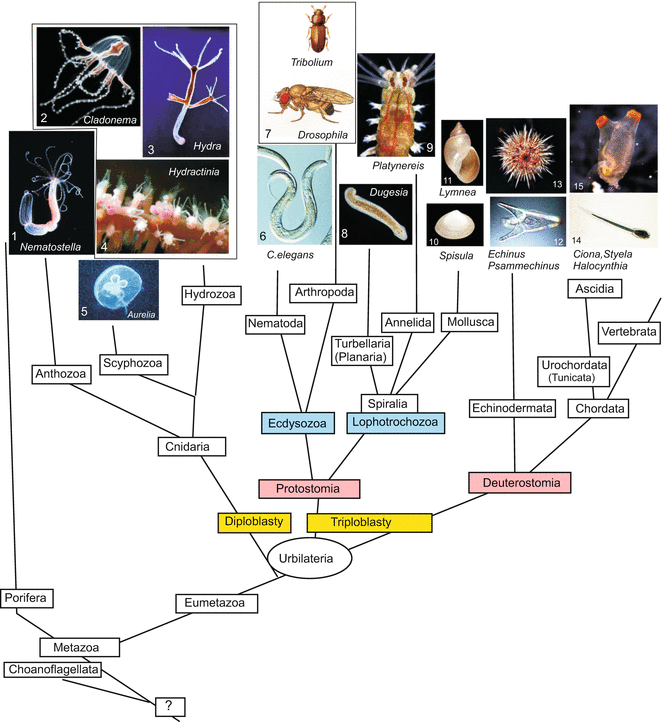
Fig. 4.1
Model organisms—invertebrates, that are dealt with or mentioned in this book, categorized according to pedigrees that are presently preferred on the basis of molecular-genetic data. Teaching picture collection of WM
4.1 Sea urchin: Basic Model of Animal Development in General and Subject of Historically Important Experiments
4.1.1 Sea urchin Gametes and Embryos Are Models of Reference for Fertilization and Early Embryogenesis
The sea urchin embryo occupies an important place in the history of developmental biology. The translucent embryos of sea urchins not only captivate us because of their beauty; in addition, they triggered many seminal ideas, and were found to be favourable material to study very early development.
As early as 1890 pioneering work on fertilization was performed with eggs and sperm of sea urchins. Even today, sea urchin eggs remain the best investigated system for studies on fertilization, egg activation and embryonic cell cycle. However, sea urchins are not good model organisms in all respects. Mutational analysis is not practical because of the long generation times and the difficulties in rearing larvae in the laboratory through metamorphosis. Mature sea urchins are not available throughout the year and must be removed from their natural marine habitat. This may pose problems with respect to species protection.
Because in the various regions of the oceans different species are found there is no single species that would represent “the sea urchin”. In littoral states of the North Sea much work is done Paracentrotus lividus and Echinus esculentus. In rim nations, Japanese and American researchers prefer to work with the Pacific species Strongylocentrotus purpuratus. In this introductory book we do not discriminate between species since species-specific differences are small in early embryogenesis. Sea urchins can make use of about 12,000 genes in controlling development, consequently the selection presented here is very restricted and determined by the current literature which focuses on homologies to developmental genes in vertebrates.
These disadvantages are balanced by several particular advantages: eggs and sperm can be harvested separately and in large amounts. Eggs are small (0.1 mm in diameter), transparent and surrounded by a translucent and easily removed envelope (jelly coat). The eggs develop in water, even under the microscope, and after artificial insemination, in perfect synchrony. Development up to the hatching larva takes 1–2 days.
Shedding of the Gametes, Fertilization.
Eggs and sperm are released through genital pores on the dorsal side of mature sea urchins. In the laboratory, sea urchins are placed upside down onto beakers, and stimulated to shed gametes by intracoelomic injection of 0.5 M KCl. Eggs are released into sea water, while sperm is shed ‘dry’ into dishes and can be used to inseminate eggs at a chosen time by mixing the milky sperm suspension into the egg suspension. The transparency of the envelope and the embryo facilitates in vivo observation of the events of fertilization, cleavage and gastrulation under the microscope.
The egg of sea urchins and the pattern of its cleavage have become prototypes in textbooks for animal eggs and development in general (Fig. 4.2). The egg is covered by an elastic, acellular coat, called the vitelline membrane, and further wrapped up in a jelly coat. In its internal structure the egg is polarized along its animal-vegetal axis. By definition, the animal pole is that pole where the polar bodies are extruded during the course of the meiotic divisions, when the egg still is in the ovary. Polar bodies are miniature sister cells of the egg cells; they will decay. The haploid nucleus of the egg is found near the animal pole. Another marker of polarity is the subequatorial band of orange pigment found in some batches of the Mediterranean species Paracentrotus lividus.
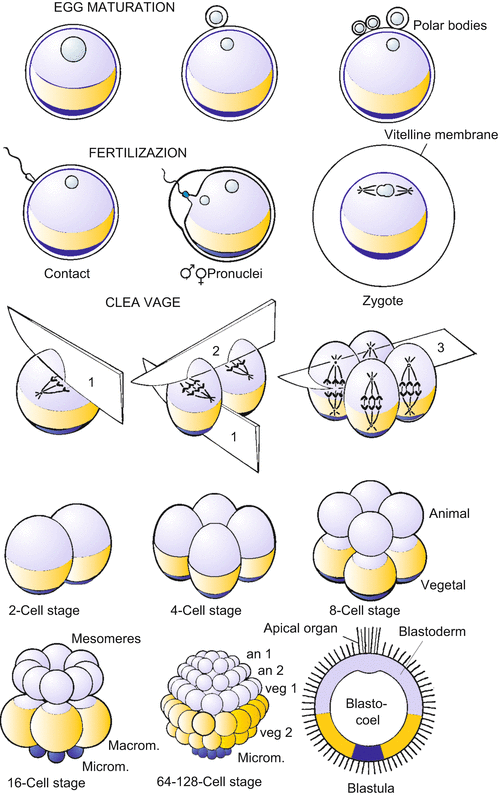
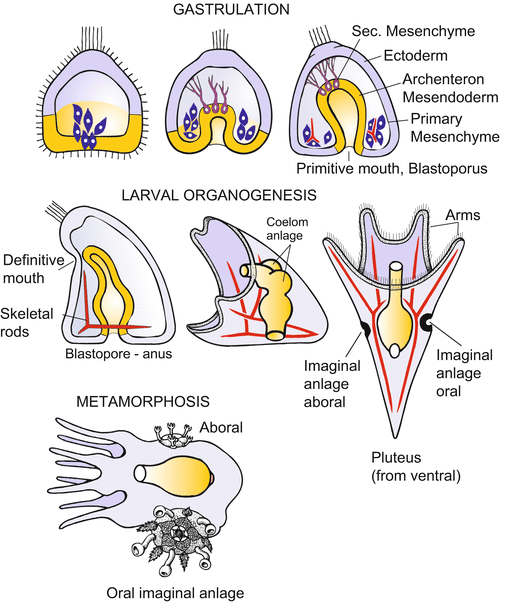
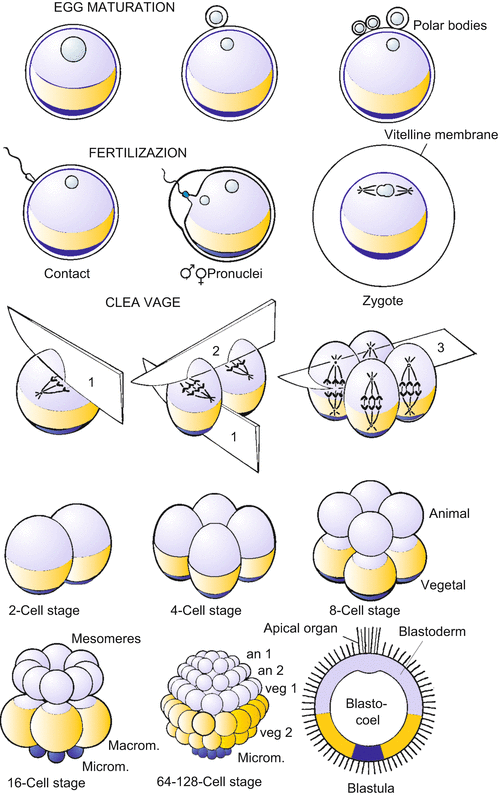
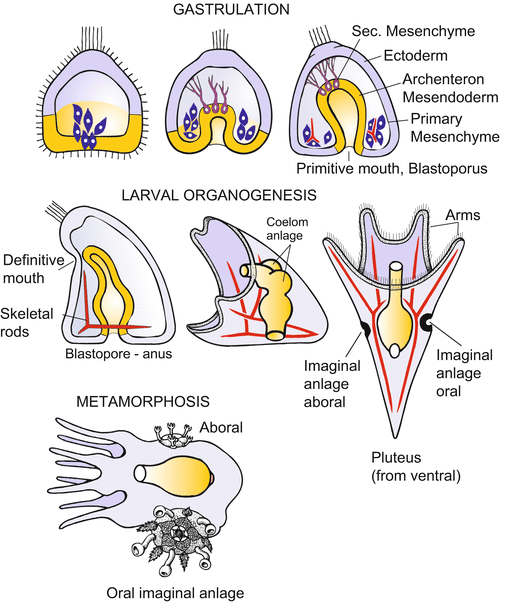
Fig. 4.2
Development of the sea urchin. (a) Cleavage: The first two cleavages pass through the animal-vegetal axis and are called meridional because they divide the egg along meridians. The third cleavage is equatorial and perpendicular to the animal-vegetal axis. Further cleavages feature oblique spindle orientation, become asynchronous and unequal, and give rise to several tiers of cells. Finally, the cells adhere more strongly to each other, form an epithelial layer, the blastoderm, and give rise to a central cavity (the blastocoel). (b) From gastrulation to the onset of metamorphosis. Gastrulation takes place in several phases and provides the interior cavity with cells from which the interior organs arise. The resulting larva is called the pluteus. From the metamorphosis of the bilaterally symmetrical larva to the pentameric adult sea urchin, only an initial stage is shown
In sea urchin eggs, unlike mammalian eggs, all of the polar bodies are formed before fertilization. Insemination, fertilization and activation of the sea urchin egg have been described in Chap. 3.
Start of Development, Cleavage.
The sperm nucleus is gated and transported into the egg. Triggered by the contact of the sperm with the egg membrane, a fertilization envelope, serving as a barrier against the penetration of further sperm, is raised from the surface of the egg, and the egg becomes activated. Following activation, cleavage divisions dissect the egg into successively smaller cells, without appreciable changes in its volume. Sea urchins undergo synchronous, radial, holoblastic cleavages until the blastula stage (Fig. 4.2a). The first daughter cells which can be individually recognized in the microscope are called blastomeres. Doubling of their chromosomes takes place rapidly, and the cells divide every 20–30 min, driven by an internal molecular clock. The embryo thus passes through the 2-, 4-, 8, -16, 32-, 64- and 128-cell stages. As development proceeds, the cell cycle lengthens and cleavage is no longer synchronous in the various regions of the embryo. A fluid-filled cavity now develops. Cells rearrange themselves to construct an outer epithelial wall enclosing the central cavity: the embryo has arrived at the blastula stage. The blastula’s wall of cells is termed the blastoderm, and its central cavity the blastocoel. On the exterior surface of the blastoderm cilia are formed; the coordinated beat of the cilia causes rotational movements of the blastula within its envelope.
At the animal pole the first larval sensory organ is formed, recognizable as a bundle of long, non-motile cilia: the apical tuft. At the vegetal pole, the blastoderm flattens and thickens to form the vegetal plate. The vegetal plate includes a group of cells, micromeres, which are filled with ribosomes. The micromeres begin to move and thereby open the phase of gastrulation.
Gastrulation begins shortly after the blastula, now comprising about 1,000 cells, hatches from its fertilization envelope. Gastrulation takes place in several phases (Fig. 4.2b).
1.
The onset of gastrulation is marked by the immigration of the descendants of the micromeres into the central cavity. The cells become bottle-shaped, release their attachment to the external hyalin layer and to their neighbouring cells, and eventually detach to migrate individually into the blastocoel. They move in the fashion of amoebae. The immigrated cells represent the primary mesenchyme. The descendants of the smallest micromeres, which were previously located around the vegetal pole, are thought to undergo terminal differentiation only after metamorphosis. But most of the cells of the primary mesenchyme soon become skeletogenic: clusters of reaggregated mesenchyme cells fuse into syncytial cables. These cables form the larval skeleton by secreting insoluble calcium carbonate that crystallizes into glittering spicules.
2.
Micromeres are emitters of inducing signals: Transplanted to another site of the blastoderm, they induce their neighbours to invaginate (Sect. 4.1.9). Invagination (Fig. 4.2b) is the mode by which the archenteron is formed in this second phase of gastrulation. The vegetal plate, consisting of descendants of the tier veg1, buckles inward and elongates within the cavity of the blastocoel to form the tube-like larval intestine. The invagination is radially symmetric around the animal-vegetal axis. When isolated surgically from the rest of the larva, the vegetal plate undergoes autonomous bending and involution.
The archenteron stretches along the length of the blastocoel. Lobe-like pseudopods on the cells at the tip of the archenteron are transformed into filamentous pseudopods or filopods. Reaching out and moving around the inner surface of the blastoderm, the filopods explore the underside of the blastoderm cells. The filopods direct the tip of the elongating archenteron to a site where the definitive mouth will later break through. The primary mouth, the blastopore, will function as the larval anus. Sea urchins and all other members of the echinoderms belong to the deuterostomes, as does our own chordate phylum.
3.
Having fulfilled their function as pathfinders, the cells at the tip of the archenteron reduce their filopodia and detach. They represent the secondary mesenchyme, which gives rise to muscle cells surrounding the gut and some other cell types.
With the ingression and involution of cells into the blastocoel, germ layer formation still is incomplete. In terms of comparative embryology the formation of the coelomic cavity is of particular interest. As the larva develops, mesoderm is formed by coelomic pouches which balloon out from the archenteron as evaginations (Fig. 4.2b). Following the segregation of the mesodermal vesicles, the remainder of the archenteron constitutes the endoderm.
Mesodermal vesicles evaginating from the archenteron called enterocoelia (see Fig. 2.2) occur in similar way also in Branchiostoma and ‘lower’ chordates, especially in tunicates. These taxa are grouped together under the term Archicoelomata. Epithelial coelomes are always considered to be derivatives of the mesoderm.
In the tradition of classic embryology the sea urchin is said to be triplobastic, meaning that it is formed by three tissue layers although three stratified sheets can never be observed. The outer layer of tissue is said to be an ectodermal epithelium, the archenteron is said to represent the endoderm and cells occupying the space between to represent the mesoderm.
4.1.2 During Metamorphosis Imaginal Discs Give Rise to the Adult Animal; the Bilateral Symmetric Larva Becomes Transformed into the Pentameric Sea urchin
Embryogenesis terminates with the hatching of the beautiful larva, which is transparent and fairy-like. It is called pluteus. The larva floats through the water and uses its cilia to swirl microscopic food into its mouth.
The transformation of the free swimming (planktonic), bilaterally symmetrical larva into the pentameric sea urchin requires a fundamental reconstruction. The new construction starts from groups of cells that were set aside from participation in embryogenesis itself. The process resembles metamorphosis in “holometabolous” insects such as butterflies, and the groups of cells that build up the new body are termed imaginal discs in sea urchins as well as in insects. The complicated, ‘catastrophic’ metamorphosis will not be described here. Experiments with embryos of sea urchins end with the observation of the pluteus larva.
4.1.3 Landmark Experiments 1. Embryos Are Not Machines and Are Capable of Regulation
The following experiment of historical significance was performed by Hans Driesch at the Stazione Zoologica in Naples. If the first two blastomeres are separated from each other at the two cell stage, each blastomere gives rise to a complete larva. Although reduced in size to half of the volume of a normal larva, both larvae are harmoniously diminished (Fig. 4.3). They are identical, monozygotic twins. If the blastomeres are separated at the 4-cell stage, quadruplets are formed. Even blastulae can be bisected, resulting in identical twin plutei, provided the embryo is bisected before the beginning of gastrulation and the cut is performed along the animal-vegetal axis. Driesch concluded that living organisms are not mere machines, because pieces of machines cannot supplement themselves autonomously to restore the complete machine. This interpretation was in striking contrast to the prevailing view of the mechanicists (Chap. 1, Box 1.1). Biologists now understand that regulation is possible because all cells are provided with the whole set of genetic information and because both halves receive animal and vegetal cytoplasmic components when the cut is made along the egg axis.
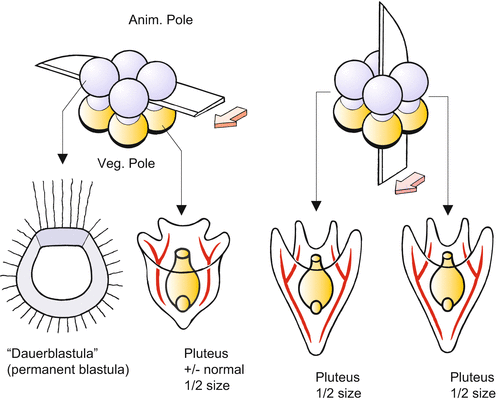
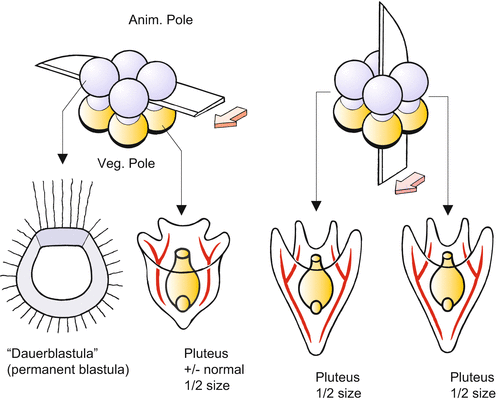
Fig. 4.3
Experimental bisection of an early sea urchin embryo at the 8-cell stage. (a) Bisection perpendicular to the animal-vegetal egg axis in the equatorial plane results in two unequal embryos. (b) Bisection along the animal-vegetal axis results in two normal larvae of half the usual size. Experiments made by Driesch (1892) and Hörstadius (1935)
From the 8-cell stage on, it is technically possible to bisect the embryo in the equatorial plane at a right angle to the egg axis. Now the result is different: The animal half develops into a blastula-like hollow sphere, which, however, is incapable of forming an archenteron. Conversely, the vegetal half is able to form an archenteron, but the emerging pluteus displays considerable deficiencies. For instance, it is mouthless and has short arms (Fig. 4.3). By displacing cytoplasm through pressure or centrifugation, Theodor Boveri, Hans Driesch and Thomas Hunt Morgan accumulated experimental evidence suggesting that cytoplasmic components are responsible for the different developmental potentials. Driesch furthermore concluded that the future fate of a cell, its “perspective significance”, is “a function of its position in the whole”. Boveri launched the idea of graded potentials along the animal-vegetal axis. The graded differences of the cells along the animal-vegetal axis were in the focal point of the following experiments.
In today’s terms in the embryo of sea urchin an early regionalization takes place that subdivides the sphere of the blastula into zones with different developmental potentials. As predicted by the founders of the experimental developmental biology maternal factors deposited in the cytoplasm at different locations prepare a first regional subdivision of the blastula. Thereafter exchange of signals between the cells make behaviour according to the local position possible. Today one speaks of positional information (Chaps. 9 and 10). For the transmission and spreading of signals gradients in the concentration of diffusible signal molecules are thought to be of particular significance. This has been deduced from the following classical experiments.
4.1.4 Landmark Experiments 2: Experimentally Deduced Interactions of Embryonic Parts Provided Reasons to Propose the Gradient Theory
Among the most remarkable experiments in the history of embryology were those performed by Sven Hoerstadius investigating the developmental potential of cells along the animal-vegetal axis of the early embryo. He separated tiers of cells from cleavage stages by transverse cuts, and juxtaposed tiers of cells taken from different locations along the animal-vegetal axis. To explain his results, he advanced the gradient theory (first formulated by Boveri). The experiments are described here in some detail because they document the scientific approach of the classic developmental biology and prompted similar experiments in many animal systems (Fig. 4.4).
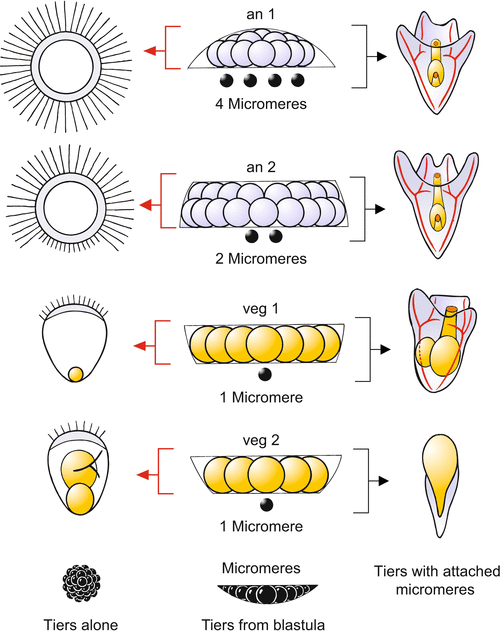
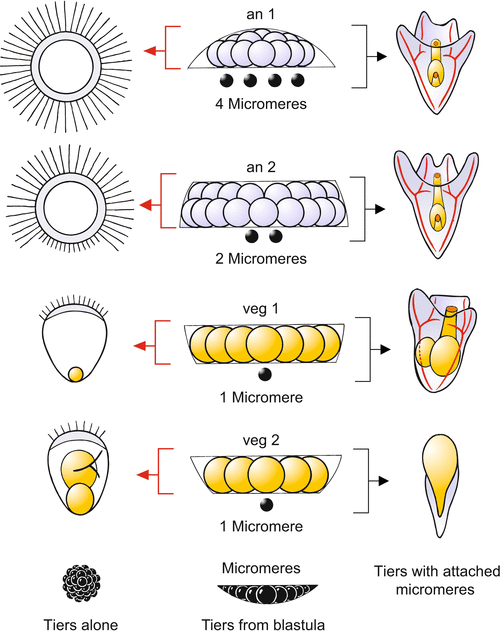
Fig. 4.4
Sea urchin. Classic experiment supporting the gradient hypothesis [conducted by Sven Hörstadius (1935, 1939)]. From middle to the left column: Tiers of cells from a 60-cell stage blastula were isolated and their developmental potentials observed. For example, tier an1 gives rise to an “animalized” blastula with an expanded apical tuft of long cilia covering the entire surface. Tier veg2 gives rise to incomplete larvae lacking the oral arms and the mouth but with an enlarged hindgut; they show features of “vegetalization”. Isolated micromeres are unable to continue development. From middle column to right column: Development of the same tiers following addition of micromeres. If 4 micromeres are added to an1, the enhanced animal potential is compensated and a normal pluteus results. To normalize an2, it takes only 2 micromeres. Addition of one micromere to veg2 leads to a highly “vegetalized” development: the ‘larva’ lacks all oral structures and the enlarged archenteron cannot be incorporated into the interior. Such a malformation is called an “exogastrula”
If the animal cap an1 is isolated by micromanipulation at the 64-cell stage, the cap gives rise to a hollow sphere unable to gastrulate. A close look at the blastula-like sphere reveals a strange anomaly: the long cilia which mark the first larval sensory organ (the apical organ) are not concentrated in a small area forming the apical tuft. Instead, the whole blastula bears these long cilia. Even the tier an2, whose descendants are normally equipped only with short cilia, develops, following isolation, into a blastula that is largely covered with long cilia. The ability to form an apical organ has spatially expanded. Such an exaggeration of structures characteristic of the animal sphere is called animalization. The tendency to form exaggerated animal structures diminishes with distance from the animal pole.
In the intact embryo the strong animal potency must be repelled or compensated by influences originating from the vegetal part of the embryo. These influences have been systematically investigated by Runnstroem, Hoerstadius and other Scandinavian researchers, who performed delicate transplantation studies. By juxtaposing micromeres onto isolated tiers of cells derived from the animal hemisphere, the exaggerated animal tendency can be compensated. Transplanted micromeres were by far the best vegetalizers. By determining the number of micromeres needed for compensation, one can titrate the strength of the animal potency (Fig. 4.4).
The tier an1 is normalized by adding 4 micromeres; the conglomeration develops into a largely normal dwarf pluteus. To normalize an2, two micromeres suffice. In veg1 one micromere suffices, but the embryo has such a weak animal component that the resulting ovoid larvae have short arms and show further defects. Apparently, an animal component is required for normal development. This requirement is even more apparent when the experiment is extended to veg2. The tier veg2, which ordinarily gives rise to a rudimentary, pluteus-like creature, is “vegetalized” by the addition of micromeres. It loses the ability to form arms and instead forms an intestine which is oversized relative to the rest of the body. As a result, the intestine cannot be invaginated into the interior cavity (exogastrula).
These results gave rise to the so-called double–gradient model: two physiological activities along the animal-vegetal axis occur as mirror-image gradients. The activities were assigned to morphogenic substance, nowadays called morphogens following a proposal by A Turing (Chap. 1, Box 1.1). The molecular identities of these hypothesized substances are now partly known (Sect. 4.1.6).
An “animalizing” morphogen that pushes determination toward animal properties has been thought to peak at the animal pole, while a vegetalizing morphogen pushes vegetal properties and peaks at the vegetal pole. Because the opposite morphogens neutralize one other, the strength of each factor decreases with the distance from its origin. The local developmental pattern is therefore determined by the ratio of two morphogens.
A popular hypothesis maintains that the morphogens can spread from cell to cell, freely crossing cell membranes. Alternatively, the relevant signals mediating interaction might spread only in the interstitial spaces between the cells or must be exposed on the cell surface and directly presented to neighbouring cells. In either case, the signal molecules have to be picked up by receptors. Such a view connects the classic gradient hypothesis with the new data.
The molecular identities of these hypothesized substances are now partly known as outlined in the following sections.
4.1.5 Cytoplasmic Determinants Stored in the Egg Are of Significance for the Orientation of the Body Axes; mRNAs of Transcription Regulators are Already in the Egg Present in Form of Gradients and Specify the Fate of the Region
Aiming at detecting factors controlling development that are stored in the egg, proteins have been sought possessing DNA-binding domains, and mRNA has been extracted encoding such proteins. Such proteins are candidates for factors controlling further gene activities and became generally known as transcription factors. The following picture emerged (Figs. 4.5 and 4.6):
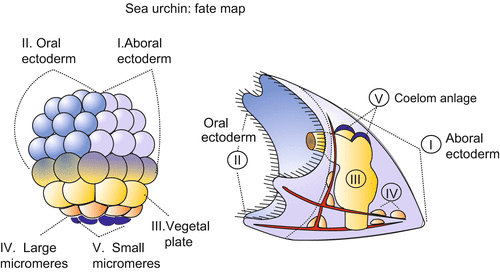
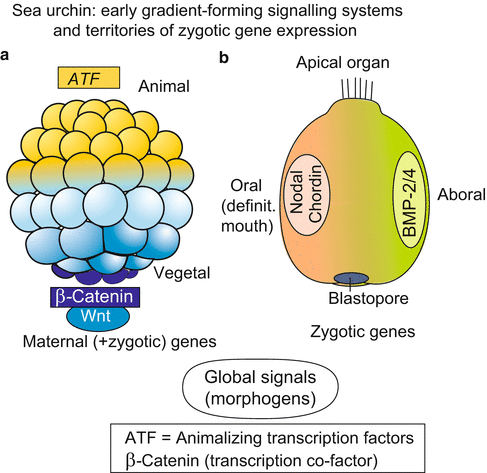
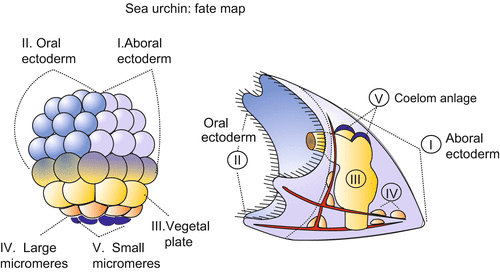
Fig. 4.5
Sea urchin: Fate map of a blastula and the corresponding structures in the pluteus larva. Various regions of the egg cell and the early blastula are equipped with different sets of maternal gene products. The different areas give rise to different parts of the pluteus larva. After investigations by Davidson
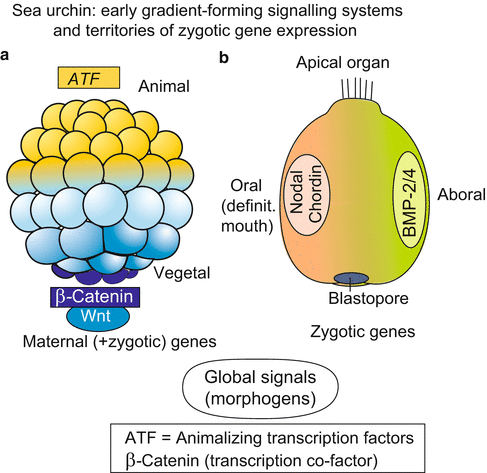
Fig. 4.6
Sea urchin, (a) Pattern of distribution of region-specifically deposited maternal gene products in the blastula. (b) Expression of zygotic genes that encode signal substances; these are also expressed in the vertebrate embryo and fulfil the criteria of antagonistically acting morphogens
As in Drosophila (Sect. 4.6) and Xenopus (Sect. 5.1) the unfertilized egg contains maternal mRNA transcribed and stored during oogenesis which codes for a large array of different transcription factors. These maternal components are deposited within the oocyte at different locations and differentially distributed among the blastomeres, but in the unfertilized oocyte wrapped in ribonucleo-protein-(RNP)-complexes. They are unwrapped after fertilization and several are found in opposite gradients along the animal-vegetal axis.
In the upper, animal half of the egg diverse ATF’s (Animalizing Transcription Factors) are found.
In lower, vegetal halve the transcription-cofactor β-CATENIN is found. In the blastula β-CATENIN has entered the nuclei of the lower hemisphere, the highest density is seen in the nuclei around the vegetal pole where the micromeres are positioned and the blastopore begins to be formed.
Beta-CATENIN is known as essential element of a signal cascade that normally starts with an externally arriving WNT signal (Chap. 11; Fig. 11.6). When the cascade is activated by WNT, β-CATENIN is taken up into the nucleus and controls gene activity in cooperation with another factor, TCF. It may be that in the sea urchin embryo maternal β-CATENIN is taken up before a WNT signal arrives as also appears to be the case in the amphibian embryo. Up to the 16-cell stage β-CATENIN is still in the cytoplasm, in highest concentration in the micromeres, and ingresses the nuclei between the 16- and 60-cell stage.
Among all the determinants identified so far β-CATENIN is the most effective one. Installed into the micromeres β-CATENIN enables them to differentiate to skeletogenic cells idependently of their environment (autonomous development) and to initiate gastrulation (Sect. 4.1.9).
β-CATENIN is also of fundamental significance for the spatial subdivision of the embryo of the amphibians and other organisms (summary in Chaps. 11 and 22; Fig. 22.6). We see a remarkable congruency. In the sea urchin as well in the vertebrate embryo and even in the cnidarian polyps the blastopore is formed in the area where stored mRNA for β-CATENIN is located (Fig. 22.7 ). In addition, the cells harbouring these factors are enabled to produce signal molecules known as inducers. These are emitted and specify the fate of neighbouring cells.
4.1.6 Long Sought Morphogens Are Being Tracked: Of Particular Significance Are the WNT-Signalling System and Signals of the TGF-β Class (BMP, Nodal) Which Are of Central Significance in Vertebrates as Well
The existence of morphogens has long been a matter of controversial dispute. Being biological signal molecules they are effective in very minute quantities and exert the expected effects only if present in the form of gradients or other spatial patterns. Gradients of morphogens have been chemically identified only a few decades ago (not until 1980) in Drosophila (Sect. 4.6) and Xenopus (Sect. 5.1).
Potential morphogens along the animal-vegetal axis:
1.
The WNT/β-CATENIN system. As just mentioned, in the blastula maternal β-CATENIN enters the nuclei and a gradient becomes established with its high point at the vegetal pole. But β-CATENIN is not an extracellular signal molecule; it cannot disperse over the boundaries of cells and consequently not act as a morphogen in terms of a signalling molecule. Yet, in the nuclei β-CATENIN switches on the gene for the WNT3a signal and this can be released into the interstitial spaces or transmitted to neighbours via transcytosis (Chaps. 10 and 11).
In this context age-old experiments have gained new interest and found a new interpretation. Sea urchin embryos that develop in sea water enriched with lithium ions become vegetalized. The expression “vegetalized” points to increased expression of vegetal morphological traits: the oral arms become too small, the intestine too large. Animal halves of blastulae are normalized not only by adding micromeres but also by bathing them in solutions of lithium-ions, and in high concentrations form even exogastrulae the large intestine of which extrudes from the gastrula instead of being invaginated.
Treatment with lithium is known to simulate the arrival of a WNT signal by interfering with signal transduction. It inhibits the enzyme GSK-3, a member of the cascade, and thus facilitates the uptake of β-CATENIN into the nucleus: Eventually WNT-dependent target genes are activated.
2.
Calcium-ions. Parallel to the gradient of nucleus-resident β-CATENIN a gradient is established in the concentration of cytoplasmic calcium-ions. Also this gradient has its high maximum in the micromeres and contributes to the specification of their fate.
3.
NOTCH system. Before the blastopore is formed the micromeres occupy the vegetal pole. They have to leave and migrate into the interior. Before they segregate and leave the micromeres talk with their neighbours by means of the NOTCH signalling system. This system has been detected in Drosophila but turned out to be a universal system of mutual arrangement if the aim is to segregate from each other. As an example the segregation of nerve cell precursors from future epidermal cell will be presented in Chap. 10 (Fig. 10.1).
4.1.7 Being a Bilaterally Symmetric Organism the Sea urchin Embryo Requires a Second Polarity Axis, an Oral-Aboral Axis. For Its Establishment the Morphogen NODAL Plays a Decisive Role
The adult sea urchin displays a pentameric radial organization but the pluteus does not; it is bilaterally symmetrical. In the structure of the egg only the animal-vegetal axis is anticipated. At the lower end of this primary body axis the blastopore is formed. In the gastrula the tube-like archenteron bends with its tip towards the outer body wall. At the position where the tip of the archenteron touches the wall the definitive mouth is formed. Now a second axis can be recognized: an oral-aboral axis which extends in about the perpendicular direction to the primary axis.
Already in the early blastula the future oral field is marked when the expression domain of the gene nodal is made visible with the technique of in-situ-hybridisation (Chap. 12). When the expression is suppressed no mouth will appear. The protein NODAL coded by the gene nodal is a member of the TGF-β family of signal molecules known from vertebrates as is BMP which becomes expressed at the opposite site of the blastula. We will meet NODAL and BMP again in the vertebrate embryo where they play indispensable roles in the establishment of the dorso-ventral body axis.
The nodal mRNA is not yet present in the egg as a maternal transcript. Which factors determine that the nodal gene is switched on in the oral field? The determining elements are factors of quite different quality and nature. In the region of the future oral pole more mitochondria have accumulated than at the opposite pole. The high oxidative activity, it is assumed, causes the switch of the gene nodal (and of several other genes) to be shifted to the “on” position. NODAL in turn switches on the gene for the protein CHORDIN, and we arrive at the
BMP/CHORDIN System.
Transcripts (mRNA) have been found for signal proteins of the CHORDIN-class at the oral pole and for a protein with high similarity to BMP2/4 at the opposite aboral pole (Fig. 4.6). Here we see a further remarkable parallel to vertebrates. In the amphibian embryo we will get to know two antagonistically acting morphogens which radiate from the ventral or dorsal site, respectively, and exert antagonistic functions as they might do in the sea urchin. In the amphibian embryo these are the morphogen BMP-4, a member of the TGF-β family, that becomes secreted in the ventral region of the blastula/gastrula, and CHORDIN that appears in the dorsal region. Emanating from opposite sites these two morphogens form concentration gradients running and decreasing in opposite directions (Figs. 5.19 and 10.4). CHORDIN binds and inactivates BMP. In the sea urchin, however, CHORDIN appears not to be of significance for the specification of the oral-aboral axis, and the definitive mouth is formed in the NODAL domain, in the amphibian it is formed in the BMP domain. In both groups of animals CHORDIN is responsible for an initial specification of the nervous system.
4.1.8 Eventually the Embryo Is Subdivided into Territories in Which Different Gene Activities Give Rise to Different Tissues and Organs
The primary gradients cease to have effects on the global body pattern after the sixth cleavage when the embryo has reached the 64-cell stage. The blastula is now subdivided in five territories that are differently supplied with transcription factors (Figs. 4.5 and 4.6).
1.
A first territory is directed by local determinants to form the ectodermal epithelium of the mouth region and the ciliated band that will extend along the arms.
2.
A second territory will form the aboral, cilia-free ectoderm.
3.
The third territory, consisting of the subequatorial vegetal plate, gives rise to the archenteron and its derivatives (e.g. the epithelium of the coelom and the secondary mesenchyme). Surprisingly, this region expresses a gene homologous to the gene brachyury that previously had been found to be expressed first in the entire mesoderm and eventually in the precursor cells of the notochord of chordates. The Echinodermata and Chordata once arose from common ancestors but echinoderms don’t have a notochord.
4.
The fourth territory defines those cells that later construct the larval skeleton.
5.
The fifth territory comprises the small micromeres which initiate the formation of the archenteron as specified in the following section.
4.1.9 Micromeres Give Origin to Short-Range Inductive Signals Which Trigger the Formation of the Archenteron
The micromeres are not only the source of morphogens that extend over long distances. Shortly before or while they migrate into the interior of the blastula they give the order for the neighbouring vegetal plate to bulge inwards to form the archenteron. When micromeres are transplanted to a different (ectopic) site they induce the formation of a second archenteron (Fig. 4.7). We will find comparable phenomena in the amphibian embryo. One speaks there of organizer effects, and similar terms are used here if the equivalence of the two events is emphasized. In vertebrates as well as in sea urchins the establishment of the organizer is associated with the uptake of the transcription factor β-CATENIN into the nuclei of the organizer cells. In the case of the sea urchin, however, the molecular identity of the signals emitted by the organizer is still unknown.
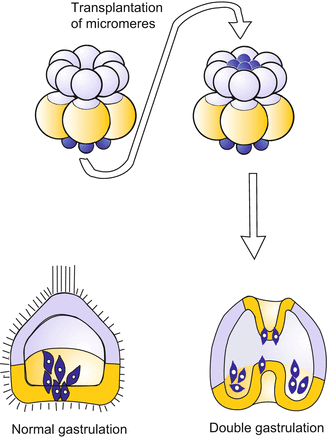
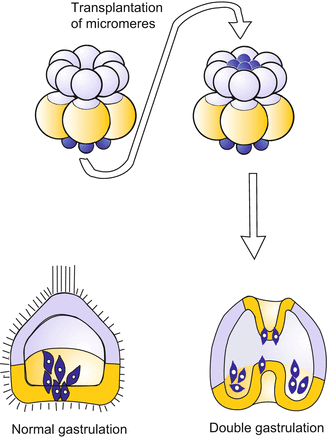
Fig. 4.7
Sea urchin: Induction of a second archenteron after transplantation of additional micromeres onto the animal pole of a blastula
4.1.10 The Ratios in Which Different Cell Types Are Represented Is Subject to Control; Lateral Inhibition Is Involved
When in the late blastula the micromeres that later would secrete the skeletal spicules are removed, the cells of the tier veg2 which normally participate in the formation of the archenteron step into the breach and take over the task of the lacking micromeres. Viewed the other way round, if enough skeletogenic micromeres are present they prevent the neighbouring future intestinal cells from fulfilling their potential for producing spicules.
However, the maternal transcription factors that have been allocated to the various territories do not enable autonomous development (except in the 5th territory). Rather the cells of the various territories exchange signals, leading to a sequence of inductive interactions. For example, by presenting signal molecules the micromeres induce, in the neighbouring tier veg2, enhanced expression of some genes and repression of others, leading to the suppression of the most vegetal developmental potency in veg2. In more general terms, by the mutual exchange of signals, the state of determination experiences fine tuning and stabilization. The ratio in which the various cell types are formed is controlled. These principles are realized in all animal organisms.
4.2 An Outsider: Dictyostelium discoideum
4.2.1 Cooperation Helps Survival: From Amoeba-Like Unicellular Organisms to a Multicellular Community
Dictyostelium is a simple eukaryotic soil-living amoeba belonging to the animal group (‘phylum’) that usually is called “cellular slime moulds”. Slime moulds (Mycotozoa) are characterized by spore-bearing fruiting bodies. However, the phylogenetic relationships are still a matter of debate. The “cellular slime mould” Dictyostelium is unrelated to multinucleated slime moulds such as Physarum, nor is it a member of yeasts or filamentous fungi. Some zoological textbooks speak of Dictyostelium as a member of the “social amoebae” (Acrasiales); recent classifications prefer to speak of Amoebozoa, subgroup Mycetozoa. Genomic studies and proteome-based phylogeny place the Amoebozoa after the plant–animal split and classify them as a sister out-group to both the animals and the fungi. The haploid genome comprises 34MB and encodes 12,000–13,000 proteins.
The organism is unusual in several aspects. Under standard laboratory conditions, the life cycle does not start from fertilized eggs, but from single haploid amoebae liberated from an envelope of spores. The life cycle of Dictyostelium is shown in Fig. 4.8. Note that it is normally entirely asexual. (A curious type of sexual reproduction is known but does not occur under standard conditions: Two cells fuse and enlarge to a giant cell by cannibalistically engulfing neighbouring amoebae. The giant cell encysts and undergoes later meiotic and mitotic divisions which give rise to new haploid amoebae.)
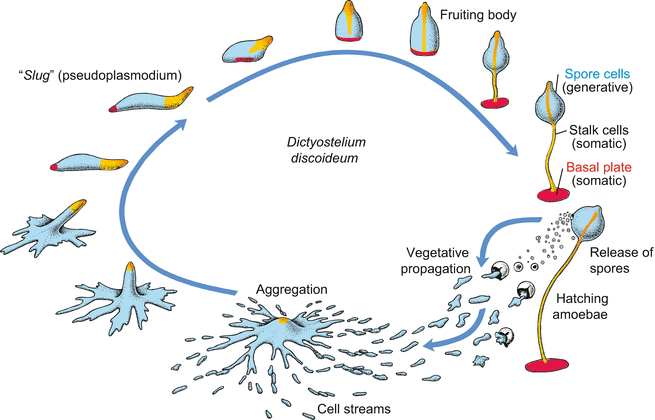
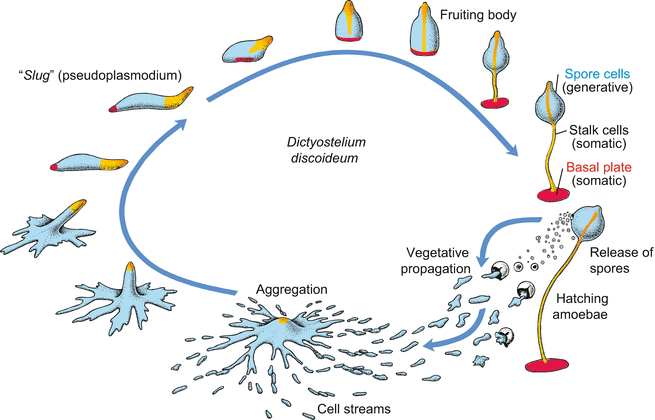
Fig. 4.8
Life cycle of Dictyostelium discoideum. Haploid cells hatch from the spore envelope, live as soil amoebae and reproduce asexually by mitotic cell division. As the food supply is exhausted, the amoebae present within a certain area assemble at a point into an aggregate. The size of the area is determined by the range of chemotactic signals emitted by the cells in the centre of the aggregate. The aggregate takes the form of a slug, migrates to an appropriate place and forms a fruiting body that releases new spore cells. (After Gilbert, Developmental Biology, redrawn and modified)
During the vegetative life cycle, an unusual transition from the unicellular to the multicellular state takes place: Numerous individual amoebae assemble to form a social community capable of making provisions for unfavourable environmental conditions. Dictyostelium has been said to be a ‘part-time multicellular organism’ formed from previously independent unicellular amoebae.
Dictyostelium lives in rich organic soils and decaying leaves. When moistened, spores seeded by fruiting bodies release haploid cells that exhibit the appearance and mode of living of amobae. They live in films of water, eating bacteria and multiplying by binary fission (vegetative phase). Only when their food supply is exhausted, or the substrate is exposed to the risk of drying out, do hundreds to thousands of amoebae assemble, migrating singly or collectively like caravans, towards a common meeting place. The aggregate absorbs all of the converging streams of cells. Eventually the aggregate forms a multicellular association that eventually acquires the form of a slug. Correspondingly, the association is colloquially called slug. In scientific terms, the association is called grex or pseudoplasmodium. The slug is surrounded by a slimy acellular sheet and is able to move much like a true slug. It migrates to a bright location where it transforms into a fruiting body, consisting of a basal plate and a stalk that bears a spherical accumulation of new spores beneath its tip. The basal plate and stalk consist of somatic cells. The somatic cells form walls made of cellulose, and eventually die. By contrast, the spore cells survive; they are generative cells whose formation and release perform the functions of asexual reproduction. Thus, the fruiting body fulfils the criteria defining a true multicellular organism: Generative, potentially immortal cells segregate from mortal somatic cells, and there occurs division of labour among the somatic cells.
Although the life cycle of Dictyostelium is not at all typical for multicellular animals (in contrast to the life cycle of sea urchins) several features of “Dicty”(lab slang) development can be taken as paradigms for similar events in higher eukaryotes. These include: aggregation, cell differentiation, and pattern formation, the spatial order in which the various cell types occur.
4.2.2 In Aggregation Pacemaker Cells in the Meeting Place Rhythmically Emit Chemotactic Signals (cAMP); These Are Strengthened by Amoebae in Their Environment and Relayed in the Periphery
Approximately five hours after removal of the food supply, hungry cells emit an attractant chemical serving to guide nearby starving cells to a central location. In Dictyostelium discoideum the chemical signal is cyclic adenosine monophosphate (cAMP). cAMP is emitted by the starving cells in synchronous pulses every 5–10 min, and spreads by radial diffusion in the water film. The signal is detected by a surface receptor protein having seven transmembrane domains like many receptor proteins in animal cells. The receptor is coupled to a signal transduction system that uses elements known from animal cells and human leukocytes, in particular elements of the adenylate cyclase-PKA system (Fig. 11.5) and phosphatidylinositol lipids. Two of them, namely phosphatidylinositol (1,4,5)-trisphosphate IP3 and phosphatidylinositol (3,4,5)-trisphosphate PIP3 are liberated from the cell membrane and used as intracellular messengers (Figs. 4.9 and 4.10) (More about signal-transducing systems can be read in Chap. 11). The responses of the amoebae include the production and release of cAMP molecules by the receiving cell. Neighbouring amoebae that have received a signal at their surface receptors respond to it by releasing cAMP of their own.
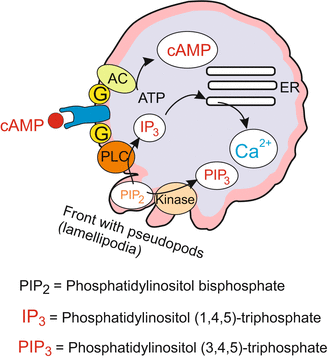
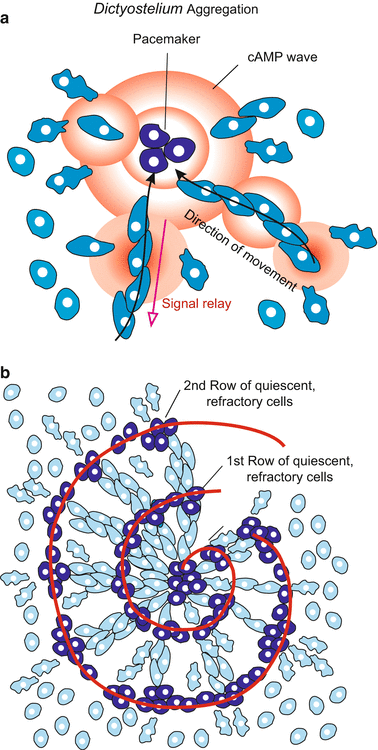
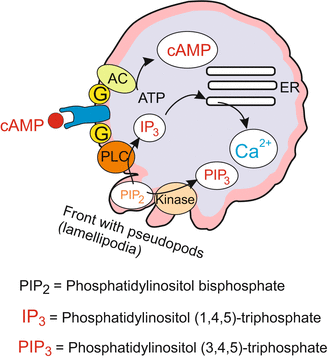
Fig. 4.9
Dictyostelium. Extracellular and intracellular signal molecules that are of significance for chemotactic orientation and migration towards the aggregation centre
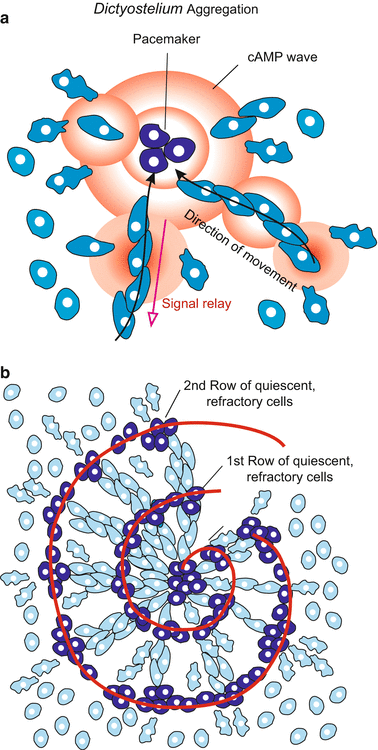
Fig. 4.10
Dictyostelium: Aggregation. (a) Amoebae in the pace maker centre (exemplified by 3 out of about 100 amoebae) synchronously emit cAMP in intervals of 2.5–5 min. cAMP signals caught by amoeba in the surroundings stimulate the receivers (1) to move in the direction of the signalling centre and (2) to emit cAMP signals themselves. While the signals spread from the centre into the periphery the amoeba move in opposite direction from the periphery towards the centre. (b) In dense aggregates spiral-shaped figures form. In the light of a phase contrast microscope momentarily quiescent cells contrast as dense clusters with stretched cells being in motion at this moment
Upon receiving a signal neighbouring cells synchronize their transmitting frequency. About 100 cells effect a hundredfold increase in the signal strength. Such a contingent of synchronized emitters becomes the pacemaker. Synchronization serves to increase the speed and range of diffusion of the released extracellular cAMP.
Nevertheless the signalling range would be restricted had D. discoideum not invented a second, highly efficient trick. To further speed up the propagation of the signal and to increase the range of signalling, D. discoideum has evolved a courier system for relaying the signal. After having received a signal each cell becomes an emitter itself. Thus, the local cAMP concentration is increased, the signal is amplified and diffusion accelerated. To ensure that the signal is transmitted from the pulsing central pacemaker to the periphery of the aggregation field, but not backward, each amoeba is unresponsive (refractory) to a further cAMP pulse for about 3 min. The transitory “deafness” prevents the cells from being disturbed by their own signal, or that emitted by their neighbours located farther from the meeting place. Only when the signal has reached inaudible remoteness are the amoebae sensitive to a new signal arriving from the centre.
Each pulse of cAMP induces a jerky movement toward the centre. As each wave arrives, the amoebae take another step towards the central meeting point. Although each cell of the population can approach the meeting place independently, they usually migrate with a caravan. Cells join with each other to form streams, the streams converge into larger rivers of cells, and eventually they all merge at the centre. The final aggregate may comprise up to 100,000 cells.
In dense aggregations often charming spiral patterns can occur. Cells being at a given moment refractory, immobile and spherical scatter light differently compared to long-stretched, moving cells. The refractory cells can arrange themselves in spiral tracks (Fig. 4.10). By developing partial differential equations to describe the spreading of the cAMP waves and the responses of the cells, mathematically talented scientists succeeded in simulating these patterns on the screen of their computer. Computer simulations are a special field of modern developmental biology (Chap. 10, Box 10.2)
4.2.3 In the Course of Aggregation Cell Differentiation Takes Place That Requires Communication and Agreement
During the aggregation phase which ends in the formation of the “slug”, the genetically uniform cell population segregates into several subpopulations: prestalk cells, representing about 20% of the population and positioned at the tip of the migrating slug; prespore cells; and the cells of the future basal plate, cells which resemble the stalk cells but form the rear of the slug.
Two hypotheses have emerged regarding the division of the amoeboid cell population into prestalk, prespore, and basal plate cells:
(a)
The positional information hypothesis: the location of the cell within the slug determines its fate.
(b)
The sorting out hypothesis: cells differentiate before or during aggregation and seek out their place within the slug according to their future role.
Both hypotheses must explain how a characteristic ratio is achieved in the numbers of the three cell types. The ratio is restored after experimental manipulation such as bisecting the slug. Surgical removal of either anterior or posterior cells causes the remaining cells to revise their developmental commitment. Whichever cells find themselves at the anterior end will become stalk cells, whichever are in the posterior region will become spore cells or basal plate cells. Eventually prespore cells and prestalk cells are present in normal ratio (Fig. 4.11). Numerical regulation is possible until cell differentiation has become irreversible in the developing fruiting body. Much evidence favours hybrid hypotheses that combine features of both positional information and sorting out.
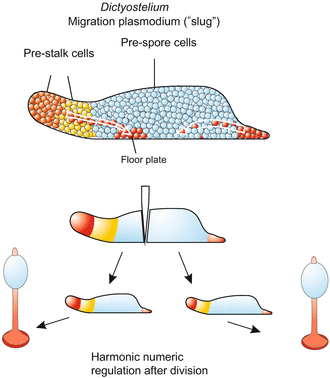
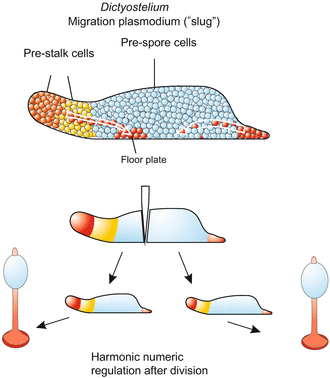
Fig. 4.11
Dictyostelium. Position of the future stalk and spore cells in the migration plasmodium, the “slug”. Upon early division of the plasmodium numerical regulation of the ratio of spore cells to stalk cells is possible
Initiation of aggregation appears to require, besides starvation, the accumulation of a secreted protein in the cell’s environment. The concentration of protein may be an indirect measure of cell density (quorum sensing), so that development only starts if sufficient cells are present to form a fruiting body. Experimental evidence also emphasizes a pivotal role for low molecular weight signal substances in the numerical control of cell differentiation and in positioning of the various cell types. Thus, cells are committed to become stalk cells in the presence of high concentrations of cAMP and differentiation inducing factor (DIF), but low concentrations of ammonium (NH 3 ), conditions that are thought to exist at the tip of the slug.
The choice between prestalk and prespore differentiation is critically dependent on the concentration of DIF. DIF is a chlorated aromate, the nucleus of which is a phenol moiety [1-(3,5-dichloro-2,6-dihydroxy-4-methoxy-phenyl)-hexan-1]. Like cAMP, DIF is liberated from starving and aggregating amoebae. NH3 is formed when surplus protein is catabolized, for instance in future stalk cells, which synthesize the N-free cellulose of their rigid and enduring cell wall before they die. The conversion of amino acids to cellulose releases ammonium.
There is evidence that additional low molecular weight substances are released to convey information in the transactions that are negotiated among the cells of the community. For instance, the nucleoside adenosine is produced from cAMP at the tip of the migrating slug and inhibits the formation of spore cells at the place of its origin. Evaporation of ammonium at the tip of the slug, on the other hand, favours the formation of stalk cells there. Evaporation is facilitated at the elevated tip. Thus, both chemical and physical conditions determine the mode of cell differentiation and the location where a particular cell type will arise.
In sum, Dictyostelium first has become a model to study periodic emission of signals, signal relay, chemotaxis and the establishment of cell contacts through cell adhesion molecules. In recent studies aspects of cell differentiation and pattern formation prevail.
4.3 The Immortal Hydra and Further Cnidaria, and the Dawn of Modern Experimental Biology and Stem Cell Research
4.3.1 Systematically Planned Regeneration Experiments With Hydra in 1740 Heralded Experimental Developmental Biology
Regeneration and grafting studies on the small freshwater polyp Hydra, performed under a magnifying glass with forceps and scalpels by the Swiss scholar Abraham Trembley 20 years before Mozart was born, rang in the era of modern experimental biology. Trembley had a green hydra fished from the Lake of Geneva. To test whether it was a plant or animal he cut the unknown creature with a scalpel in pieces. In those days European scholars were familiar with ancient Greek literature, and following Aristotle thought that only plants were capable of regeneration. Although he observed an incredible power of regeneration he eventually concluded that the creature was an animal. (It was Linneus who later named it Hydra with respect to the ancient water monster who quickly replaced each cut off head with two). Trembley’s observations are well documented in detailed descriptions and masterful engravings. Two and a half centuries later, developmental biologists still make use of the enormous capacity of reconstitution and regeneration displayed by Hydra and its marine relatives. Hydra readily replaces lost body parts – head, foot, or any other part of its tube-like body.
Regeneration studies are supplemented with transplantation experiments (grafts) like those shown in Figs. 4.15 and 10.15. Tissue grafts performed by the American Ethel Brown led in 1909 to the discovery of the phenomenon of induction; today also known as organizer activity (Chap. 10).
However, Hydra is not always co-operative. It is not a useful model to study early embryogenesis from an egg, and a classical genetic approach is very tedious if not impossible. As a rule, gametes are produced only under adverse and stressful environmental conditions, and only in small numbers. The eggs are enclosed in a rigid, non-transparent envelope. The investigator interested in embryogenesis (from the egg to the planula larva) or metamorphosis (from the planula to the polyp) should consider several marine relatives (Sect. 4.3.7).
4.3.2 Hydra Represents an Ancient Metazoan ‘Bauplan’; Yet, the Cellular Composition of Its Body Is in a Permanent Steady State and the Body Is, Thanks to Stem Cells, as a Whole Potentially Immortal
The tube-like body (Fig. 4.12) displays a unipolar, radial organization with a hypostome encircled by tentacles (colloquially called the “head”) at the upper end of the body column and an adhesive basal disk (“foot”) at the lower end. The opening at the upper pole serves as mouth through which prey (mainly small crustaceans caught with the surrounding tentacles) is engulfed (or as an anus through which remnants are expelled). The body wall consists of two epithelial sheets, ectoderm (epidermis) and endoderm (gastrodermis); Hydra is said to be diploblastic in contrast to the triploblastic bilaterians which all develop from embryos with three germ layers. The genome of Hydra comprises about 20,000 protein-encoding genes.
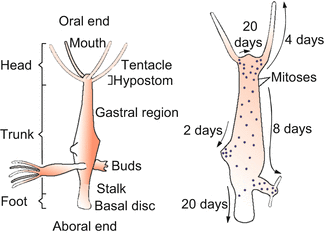
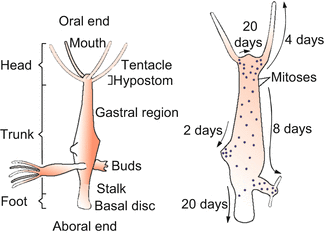
Fig. 4.12
Hydra with buds. Right: Dots along the animal indicate sites of cell division; the arrows indicate the direction in which the epithelial cells are displaced. The shift of epithelial cells results from two events: (1) In the terminal body regions used up or aged cells undergo cell death and are phagocytosed or sloughed off, while (2) new cells are born in the body regions between the terminal regions. A surplus of cells is exported in the form of buds. Once a bud has formed a head and a foot, it detaches to live as a new, independent individual. It is genetically identical with its parent (it is cloned)
Hydra is member of the phylum Cnidaria: the simplest metazoans (multicellular organisms) possessing typical animal cells such as sensory, nerve- and epithelio-muscle cells.
The inventory of cell types is compiled in Fig. 4.13. Traditionally the cells of a hydra are subdivided into two main categories:
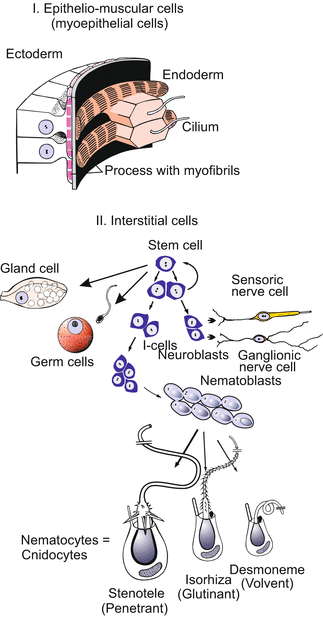
1.
Epithelial cells determine the basic architecture of the body. Two adjoined epithelial layers, the ectoderm and the endoderm, form the walls of the tube-like body. As these cells also serve as muscle cells mediating the movements of the body column they are also called epithelial-muscle-cells.
2.
Interstitial cells occur in spaces (interstitia) between the epithelial cells. Interstitial cells include sensory nerve cells, ganglionic nerve cells, four types of stinging cells, a type of gland cell, gametes, and the stem cells of all these cell types.
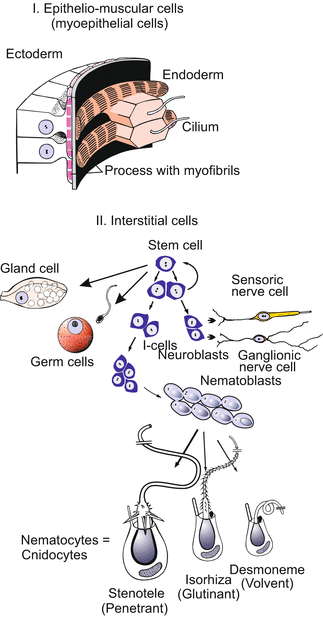
Fig. 4.13
Hydra. Basic cell types of a hydra. Diagrammatic presentation; sizes are not to a consistent scale. In the ectoderm, muscle fibres run longitudinally and mediate the contraction of the body column; in the endoderm muscle fibres run circumferentially and mediate the expansion of the body column. Derivatives of the interstitial stem cells reside in the spaces between the epithelial cells (except the gland cells that become integrated into the endodermal epithelium, and the mature nematocytes that are mounted within ectodermal ‘battery cells’ of the tentacles)
Despite being equipped with mortal cells such as nerve cells Hydra as a whole is potentially immortal. Immortality is achieved by an unlimited capacity for self-renewal: the polyp can replace any aging cell, or cells having fulfilled their tasks, by substitutes generated from immortal stem cells. In this process of perpetual renewal all cells are replaced even the nerve cells. Cell proliferation, cell differentiation and cell migration never come to a standstill. The hydra is a perpetual embryo, and although its terminally differentiated cells die, the cell community survives.
According to the cellular inventory, three categories of stem cells are distinguished in the genus Hydra. 1st Ectodermal epithelial stem cells, 2nd endodemal epithelial stem cells, 3rd pluripotent interstitial stem cells (abbreviated i-cells). The interstitial stem cells give rise to the interstitial cell lineages: nematocytes, sensory and nerve cells, gland cells and gametes.
The epithelial cells, even those of the head and the foot, arise from epithelial stem cells in the middle of the body column. These cells are not stem cells in the conventional sense because they are differentiated; but they retain the capability of dividing and self-renewal.
The cells born in the middle of the body column are displaced towards the mouth; they become integrated into the growing tentacles or into the mouth cone. Other cells are shifted towards the foot. Having arrived in the terminal body regions (in the mouth cone, the tentacles or the foot), they undergo terminal differentiation and eventually die. They are sloughed off or phagocytosed by neighbours. As a hydra has no defined lifetime this steady state of production and loss of tissue goes on continuously when the animal is fed regularly.
In well-fed polyps a surplus of cells generated in the gastric region is used to produce buds and thus the surplus is exported. Buds detach as self-reliant animals. By budding, Hydra clones itself.
4.3.3 Hydras That Lose Their Nerves Survive
Hydras can be deprived of their interstitial stem cells by treatment with chemicals (hydroxyurea) or, in a particular sensitive strain, by a transient increase of the temperature. As a consequence, the animals can no longer replace used up nematocytes and aged nerve cells. Astonishingly, if artificially fed on a regular basis the animals survive in spite of becoming, in the long term, deprived of their entire nervous system. Nerve-less hydras can regenerate their head and foot, and even produce nerve-free offspring by budding, and a clone of nerve-less hydras can be established. When new stem cells are introduced through transplants new nerve cells and nematocytes occur and the polyps regain a normal appearance and behaviour.
4.3.4 Hydras Can Be Dissociated Into Single Cells; a High Capacity for Self-Organization Enables Aggregates of Such Cells to Give Rise to Whole Animals
Astonishingly: The hydra polyp can be dissociated experimentally into single cells, which sink to the bottom of the dish, creep around like amoebae, re-establish contact with one another and form aggregates of cells, called re-aggregates. Initially, re-aggregates are disorderly arranged clumps of cells and inconspicuous. But within days or weeks, the aggregates organize themselves to form new, viable polyps. Reorganization takes place in steps.
Sorting Out.
First ectodermal and endodermal cells segregate. Amoeboid movements of cells and selective cell adhesion enable and cause endodermal cells to form an inner ball-like mass that becomes enveloped by an extending sheet of ectodermal cells. Eventually a blastula-like hollow sphere is formed with an endodermal inner and an ectodermal outer layer.
Pattern Formation.
Following the reconstitution of an epithelial organization a process of pattern formation takes place, beginning chaotically and ending with order (Fig. 4.14). Bunches of tentacles emerge in initially irregular arrangements. Mouth cones push their way between them; the more that do so the larger an aggregate is. The positions of the tentacles are shifted in circles around the mouth cones, superfluous tentacles are reduced, new ones fill the gaps. Each “head” so formed becomes the organizer of a body axis; this emerges from the aggregate and lifts up the head at its end. Eventually feet are formed and these have the faculty to segregate. From the multi-headed monster single, normally-organized polyps detach one after the other. This process of reorganization is called reconstitution.
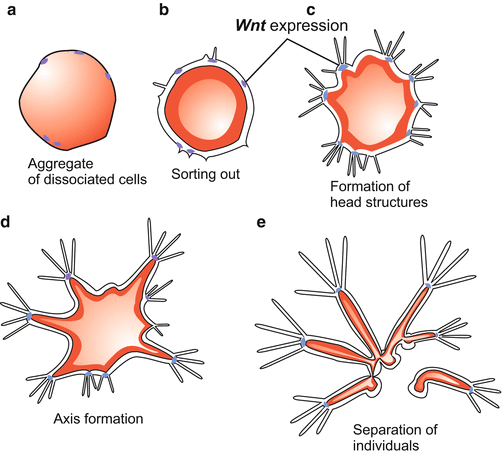
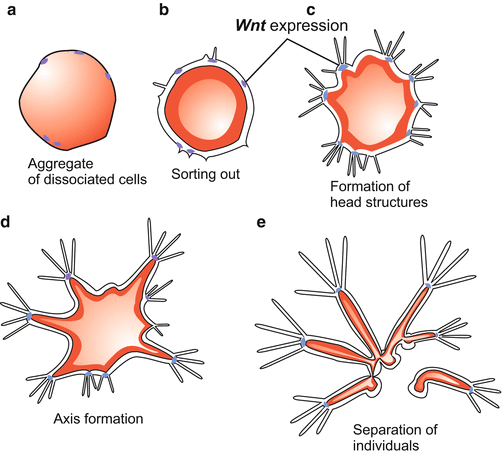
Fig. 4.14
Hydra. Reorganization in an aggregate of dissociated cells. At the end of this process that takes several days complete polyps of different sizes detach by autonomous splitting of the feet. After a preparation by the author WM. (Since the emerging polyps were fed buds also appeared which are omitted for clarity.) Wnt expression after Hobmayer et al. (2000)
4.3.5 Permanent Cell Renewal Presupposes Positional Information; This Is Analyzed in Classic Regeneration and Transplantation Experiments
Recent Hydra research has emphasized, besides self-renewal through stem cells, patterning and the regulation of cell differentiation. Pattern formation and positional information have been investigated in the context of regeneration and reorganization. Depending on where a group of cells is, they can organise themselves to form a hypostome with tentacles (“head”), gastric region, stalk, or basal disk (the “foot“) (Fig. 4.15a). But how do the cells recognize where they are? How positional information is supplied and how the body pattern is corrected after experimental interference will be discussed in Chap. 10. Here some findings are summarized.
The head functions as an organizer, comparable to the Spemann organizer in the amphibian embryo (Sect. 5.1 and Chap. 10). Small pieces taken from the head region and transplanted into the trunk influence the trunk cells in their environment in such a way that they participate in formation of a complete additional head (Figs. 4.15b, 10.19, and 10.20).Stay updated, free articles. Join our Telegram channel
Full access? Get Clinical Tree
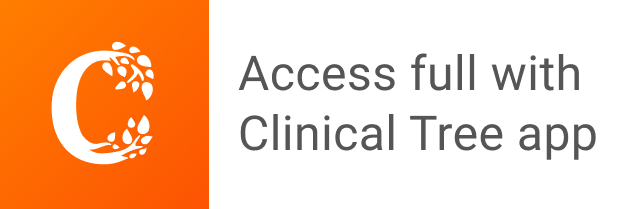