The horse was domesticated around 3000 BC, probably in what is now Southern Russia.1 From the beginning, the species had a special position. Unlike most other species that had been domesticated before, the horse was not to provide human beings with tangible products such as meat, milk or fur, but to serve mankind with its exceptionally developed locomotor system. The common denominator of all uses of the horse, whether in the past or in modern-day society, is that they are based on the species’ very well developed locomotor system that has enabled the evolution from the less than two hands tall eohippus or Hyracotherium, which was a forest-dwelling browser relying on hiding for survival, to the approximately 15 hands tall Equus Przewalski, a grazing inhabitant of open savannahs and a typical example of a flight-and-fright animal that survives thanks to its locomotor performance. Given the prominent role of locomotion for the performance of the horse, it will be no surprise that disorders of the musculoskeletal system rank first in causes of wastage in the equine species. Most figures come from the racing industry2, but also the relatively scarce data for sport horses point in the same direction.3 Of all musculoskeletal disorders, joint problems rank, depending on equestrian discipline, invariably first or second (after tendon injury) as a cause of loss of performance.2,4 They are rarely as acute and dramatic as fractures, but their insidious onset and the inherently low regenerative capacity of (mature) articular cartilage makes them a major plague to the equine industry, which has large economical impact and is a severe welfare issue as well. There is an important parallel with human medicine. The importance of joint disease in man was already well recognized in the eighteenth century when William Hunter wrote his famous statement that ‘ulcerated cartilage is universally allowed to be a very troublesome disease; that it admits of a cure with more difficulty than a carious bone; and that, when destroyed, it is never recovered’.5 Chronic joint disorders, of which osteoarthritis (OA) is the most important, are a literally crippling problem in human health care and an important cause of loss of quality of life. Directly in parallel to the equine situation, joint disease is an important issue in human sports performance and therefore there is much interest in the effects of exercise and training on joint function, an area to which relatively much research effort has been dedicated in the past decades. Where research into joint disorders has long focused on single tissues only (mainly articular cartilage), the current concept is that joints should be seen as organs where the constituting tissues (articular cartilage, subchondral bone, synovial membrane, synovial fluid and in some cases intra-articular ligaments or menisci) act together and proper joint homeostasis is the key element.6 The effects of exercise and/or training on joints can be translated as changes in (mechanical) loading of joints, which will elicit responses from the tissues and hence affect joint homeostasis. The range of effects on both sides of the optimum is wide and goes from direct trauma to stress deprivation. The almost complete immutability of cartilage, as already alluded to by Hunter, is an important and specific feature. However, in young, growing individuals the plasticity of cartilage is considerably greater and this makes that discrimination should be made between the effects of exercise in full-grown adults and juvenile individuals. The potential long-term effects of (lack of) exercise in youngsters have received relatively much attention in the past years, both in horses and humans. Figure 11.1 is a schematic drawing of a diarthrodial joint, outlining its principal components: subchondral bone, cartilage, the joint capsule and synovial fluid. These components will be reviewed briefly in the following section. The emphasis will be on articular cartilage, which is the joint tissue with most morbidity and least regenerative capacity. The subchondral bone supports the overlying cartilage and is connected with it through a layer of calcified cartilage. Typically, subchondral bone consists of a layer of compact bone directly adjacent to the layer of calcified cartilage and trabecular bone at greater distance from the joint cavity. This conformation has mechanical consequences: the compact subchondral plate provides firm support, but is rigid, while the trabecular component provides some elasticity. Sclerosis of the subchondral plate is one of the hallmarks of OA and it has been suggested that this might be a primary event in cartilage damage, rather than a sequel to it, which is the commonly held opinion.7 Subchondral bone is, unlike cartilage, richly vascularized and has a well-developed nerve supply that plays a major role in pain perception in joint diseases. The collagen fibrils consist of collagen type II molecules (3 α1 chains in triple helix configuration) and form a three-dimensional arcade network.8 The fibrils are anchored within the layer of calcified cartilage that is adjacent to the subchondral bone, then course perpendicular to this layer towards the joint surface to bend before reaching the surface, running tangentially to and directly under it, to close the arch by bending back again and returning perpendicularly to the calcified cartilage layer where they end (Fig. 11.2). Structural coherence and extra strength is provided by cross-links that connect the α1 chains within the same collagen molecule and interconnect different collagen molecules and different collagen fibrils. The formation of cross-links is one of the so-called post-translational modifications of collagen and is the last chemical modification occurring during the formation of the primary collagen structure. There are various types of cross-links. Common covalent cross-links are the pyridinoline cross-links that form between lysyl and hydroxylysyl residues in the collagen network (lysylpyridinoline or LP cross-links and hydroxylysylpyridinoline or HP-cross-links respectively) in a largely irreversible process. They have a major influence on the structural and hence on the biomechanical characteristics of the collagen network.9 A special category of cross-links is formed through the process of non-enzymatic glycation. Collagen molecules have an exceptionally long lifetime once incorporated into the ECM of cartilage, which makes them susceptible to the accumulation of advanced non-enzymatic glycation end products (AGEs) via the so-called Maillard reaction. This process results in increased cross-linking, such as pentosidine formation from lysine, sugar and arginine moieties. Pentosidine is one of the few Maillard cross-links of which the structure has been elucidated and can be used as a sensitive marker for the process of non-enzymatic glycation. As the accumulation of AGEs depends on the turnover rate of a protein or tissue, they can be used as a measure for the metabolic rate of that structure.10 Due to anatomical and geometric particularities, joints are not loaded evenly over their surface. Different areas are subject to different magnitudes and types of loading, such as low-level constant loading, intermittent loading, and very high impact loading. These variable loading conditions can only adequately be met by cartilage that possesses different mechanical properties in different sites.11 This heterogeneity in biomechanical behaviour can only be accomplished through different biochemical and ultrastructural characteristics, which indeed have been shown to exist (Fig. 11.3).12–16 Cartilage not only displays ‘horizontal’ zonal heterogeneity over the surface, but also ‘vertical’ along its depth. The superficial layer is characterized by flattened chondrocytes, densely packed type II collagen fibrils running parallel to the surface, a relatively small amount of proteoglycans (PG) and high water content.17 The middle or transitional zone has lower water content, more PG and a lower density of collagen fibrils that seem less organized because they form arches and thus do not run parallel, and rounded chondrocytes dispersed irregularly in the extracellular matrix. The deep zone has collagen fibrils that are oriented perpendicular to the articular surface (again running parallel to each other), the highest concentration of PG but the lowest percentage of water of any zone, and chondrocytes arranged in columns perpendicular to the subchondral bone. Articular cartilage may seem to be a very static tissue, but it undergoes constant remodelling through anabolic and catabolic processes. The chondrocyte, as the only cell type found in normal articular cartilage, produces all ECM components. Collagen molecules are manufactured intracellularly in the form of pro-collagen and are subsequently extracellularly modified by enzymatic cleavage of the C- and N-terminal propeptides before being integrated in the collagen network. Degradation of ECM is effectuated by various proteinases, of which the matrix metalloproteinases (MMPs) and aggrecanases (members of the disintegrin and metalloproteinase with thrombospondin motifs (ADAMTS) gene family) are the most important. It is important to note that the two principal constituents of the cartilage ECM, collagens and proteoglycans, have very different turnover rates. Turnover time of proteoglycans is variable and ranges in mature individuals from approximately 300 days for overall turnover in canine articular cartilage to around 20 years for the long-lived globular hyaluronic acid binding domain of aggrecan in humans.18 This may seem long, but collagen turnover times have been estimated to be up to 350 years in mature human cartilage.19 Comparable data for the horse do not exist, but can be assumed to be in the same order of magnitude. It is this very low turnover rate and thus extremely limited repair capacity that lies at the basis of the famous observation by William Hunter cited earlier5 and that makes OA in both humans and horses into a disease with an almost invariably poor long-term prognosis for performance. The synovial membrane has no basement membrane or tight junctions. In fact, the cells are located within a loose and porous bed of collagen fibrils and other matrix proteins. This configuration allows the diffusion of all but the largest molecules (>10KDa) from the blood to the synovial cavity and synovial fluid (SF) can hence be seen as an ultrafiltrate of blood plasma, supplemented with hyaluronic acid and other products from the A and B type synoviocytes. This situation allows the supply with nutrients and the removal of waste products from the avascular cartilage. Synovial fluid is yellowish in color and highly viscous, due to the high hyaluronic acid content. The cellular component is limited, normally not surpassing 0.5 G/L, and consists mainly of lymphocytes and other bone marrow derived mononuclear cells. The SF can be seen as a mirror of joint homeostasis par excellence, as it bathes both tissue types that line the synovial cavity, articular cartilage and the synovial membrane. In case of an infringement of joint homeostasis, the synovial membrane is likely to respond first as the most reactive of the tissues and may produce a plethora of inflammatory mediators and cytokines, of which Tumour Necrosis Factor alpha (TNF-α) and Interleukin 1 beta (IL-1β) are known to be major players in joint disorders. Through their dispersion in the SF, these mediators affect the cartilage. The determination of the inflammatory profile of SF has received increased attention in recent years in the context of the quest for early detection of OA, as it now is widely accepted that also in this seemingly ‘degenerative’ disease, low-grade inflammation plays a crucial role too.20 On the other hand, metabolic responses of the cartilage will be reflected in changes of in levels of products and by-products of proteoglycan and collagen metabolism, such as the proteoglycan marker CS846, and C2C and CPII as makers for collagen break-down and synthesis respectively.21 In theory, there are two possible origins of the topographical heterogeneity of articular cartilage: genetic predetermination or formation in the early juvenile period when cartilage metabolism is still high. The first indication for the latter option came from work by Little and Ghosh22
Joint physiology
Responses to exercise and training
Introduction
Principles of joint physiology
Subchondral bone
Cartilage
Synovial fluid
Loading the immature joint: crucial conditioning
The origins of topographical heterogeneity of articular cartilage
Stay updated, free articles. Join our Telegram channel
Full access? Get Clinical Tree
Joint physiology: Responses to exercise and training
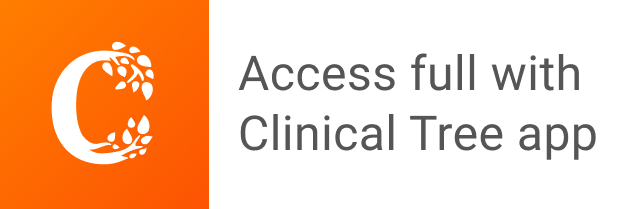