Stephanie M. Hamilton Department of Companion Animals, Atlantic Veterinary College, University of Prince Edward Island, Charlottetown, Prince Edward Island, Canada The drugs described below produce reliable sedation and anesthesia in veterinary patients. Most commonly, these agents are administered intravenously (IV) to induce an unconscious state suitable for intubation and transition to an inhaled anesthetic. However, when administered by either constant rate infusion, intermittent bolus, or intramuscularly (IM), injectable anesthetics can also be used to maintain anesthesia for short periods of time. Ideally, all injectable anesthetics would be water soluble, have a long shelf‐life, and be stable when exposed to heat and light. Only a small volume of drug would be needed to induce anesthesia, and these agents would have a large safety margin. Their duration of action would be short, with no cumulative effects, and they would be readily metabolized into non‐toxic metabolites and/or excreted from the body. Their half‐lives would be well characterized, as would their maximum residual limits so that withdrawal times could be established for animals destined for human consumption. Analgesia adequate for the procedure and some degree of muscle relaxation would also be produced by ideal injectable anesthetics. Most importantly perhaps, the ideal injectable anesthetic would not create unpredictable life‐threatening changes in cardiovascular and respiratory function. An injectable anesthetic that possesses all of these characteristics has not yet been produced. When selecting an injectable anesthetic, the practitioner should consider the pharmacokinetics and pharmacodynamics of the anesthetic, in addition to the patient’s physical status, in order to select the most appropriate agent and dose for that individual patient. Barbiturates have been used in veterinary medicine for decades as both injectable anesthetics and anticonvulsants. For many years, thiopental was among the most commonly used injectable drugs for induction of anesthesia. However, over the past decade, its lack of availability in the United States and other countries has meant that veterinarians have increasingly turned to other non‐barbiturate injectable anesthetic drugs. All of the drugs in this category are derivatives of barbituric acid, a combination of urea and malonic acid (Fig. 27.1). While barbituric acid does not have sedative or hypnotic properties, side chains added at position 5 in the pyrimidine nucleus impart hypnotic activity. The length of the side chain at position 5 influences the potency and the duration of action of these drugs, with longer side chains increasing potency. If a sulfur atom replaces the oxygen atom at position 2, an active barbiturate with a faster onset of action and a shorter duration is produced. In general, any modification of the barbiturate that increases the lipophilicity of the molecule will increase its potency while shortening the onset time and duration of action. Many barbiturates (thiopental, thiamylal, and methohexital) have asymmetric carbon atoms in one of the side chains attached to the barbiturate ring at position 5, which results in stereoisomers. Despite differences in potency of the stereoisomers (i.e., S(–)‐enantiomers are nearly twice as potent as R(+)‐enantiomers), barbiturates are supplied as racemic mixtures. Commonly, barbiturates are classified by their duration of action (long, intermediate, short, and ultra‐short) or their chemical structure. Thiobarbiturates (thiopental and thiamylal) are those with a sulfur atom at position 2, while oxybarbiturates (pentobarbital, phenobarbital, and methohexital) have an oxygen atom at position 2. Historically, the ultra‐short‐acting thiobarbiturate, thiopental, was commonly used for anesthetic induction in veterinary medicine. In jurisdictions where it is still available, it is supplied as a yellow crystalline powder buffered with sodium bicarbonate, which is usually reconstituted with sterile water or saline to produce 2.5%, 5%, or 10% solutions. The resulting solution is alkaline (pH 10–11) and can cause tissue necrosis if injected perivascularly. The reconstituted solution is stable at room temperature for up to 1 week [1]. As the solution ages, crystals precipitate resulting in a progressive loss of potency; therefore, higher doses may be needed to induce anesthesia. Thiamylal is an ultra‐short‐acting thiobarbiturate that differs from thiopental in that the ethyl radical in thiopental has been replaced with an allyl radical. While thiamylal was commonly used in veterinary medicine for some time, it is no longer commercially available. Methohexital is an ultra‐short‐acting oxybarbiturate that possesses a methyl group at the N‐1 position. This results in a drug that is twice as potent as thiopental, but also has an increased incidence of excitatory side effects. Methohexital sodium is supplied as a powder and is reconstituted with sterile water or saline to produce a 2.5% solution that is stable for up to 6 weeks if refrigerated [2]. Figure 27.1 General chemical structure of barbiturates. Pentobarbital is classified as a short‐acting oxybarbiturate that is identical to methohexital but lacks a methyl group at the N‐1 position. These substitutions result in a drug that has a longer duration of action (typically 1–2 h) and a lower margin of safety (anesthetic dose is 50–70% lethal dose). Pentobarbital has largely been replaced as a general anesthetic in most domestic species. It is still used as an injectable anesthetic in laboratory rodents, particularly in non‐recovery procedures, and remains the primary ingredient in most commercially available veterinary euthanasia solutions. Pentobarbital is available in solutions of varying concentrations that, stored at room temperature, are stable for years. Barbiturates produce central nervous system (CNS) depression by activating the ionotropic subtype of the γ‐aminobutyric acid (GABA) receptor known as “GABAA” [3]. Activation of the GABAA receptor increases transmembrane chloride conduction, resulting in hyperpolarization of the postsynaptic cell membrane. It appears that the barbiturates reduce the rate of dissociation of GABA from its receptor, which increases the duration of chloride channel opening. At increasing drug concentrations, barbiturates can mimic the action of GABA and activate the chloride channels directly [3,4]. Ultimately, inhibition of the postsynaptic neuron results in CNS depression and loss of consciousness. Distribution of the barbiturates within the body is determined by factors such as time, protein binding, degree of ionization, and lipid solubility. Lipid solubility increases with the substitution of a sulfur molecule at carbon position 2 in the barbiturate ring. As previously stated, an increase in lipid solubility increases potency and shortens onset and duration of action. Protein binding correlates with lipid solubility, with barbiturates that are highly lipophilic (thiopental) also being highly bound to protein. Decreased protein binding due to displacement from binding sites by other drugs (aspirin and phenylbutazone) or hypoproteinemia can lead to increased drug effects. The acid dissociation constant (pKa) and pH of the environment can be used to predict the proportion of ionization of the barbiturates for any given condition. The pKa is the pH at which the barbiturate exists 50% in the ionized and 50% in the non‐ionized form. For a barbiturate to penetrate the lipid layer of a cell, it needs to be in the non‐ionized form. Therefore, as blood becomes more acidic, more non‐ionized drug exists. This leads to an increase in the barbiturate’s CNS penetration and clinical effectiveness. The reverse is also true: with more alkaline blood, the ionized form is favored, and the anesthetic effect may be reduced. Metabolism of the barbiturates occurs in the liver and is followed by excretion by the kidneys. Biotransformation (oxidation, N‐dealkylation, desulfuration, and destruction of the barbituric acid ring structure) in the liver occurs primarily in the endoplasmic reticulum of hepatocytes and can result in hepatic enzyme induction (cytochrome P450 system) [5]. The reserve capacity of the liver is quite large; therefore, significant hepatic dysfunction must be present before there is a prolongation of the duration of action of barbiturates [6]. After IV administration of thiopental, the drug mixes within the blood and is delivered to body tissues in accordance with the rate of perfusion, the tissue affinity for the drug, and the relative concentration of thiopental in the blood and tissues. Well‐perfused, small‐volume tissues, such as the brain, equilibrate rapidly with the thiopental concentrations in the blood, inducing anesthesia. The concentration of thiopental in the blood and brain falls rapidly as the drug redistributes to less perfused muscular tissues, allowing the animal to regain consciousness. Dogs receiving 15 mg/kg of thiopental IV remained unresponsive to noxious stimuli (toe pinch) for 12 ± 6 min. Recovery to sternal recumbency took 52 ± 29 min and standing took place 77 ± 32 min after administration [7]. The principal factor limiting anesthetic duration after a single dose of thiopental is redistribution from the brain to other tissues [5]. Therefore, when thiopental is administered in large doses, as repeated doses, or as a constant rate infusion, recovery from anesthesia may be prolonged as lean tissues (e.g., muscle) approach equilibrium with the concentration of thiopental in the blood. This equilibrium progressively decreases the capacity of the tissues to remove the drug from the blood [8,9]. Additionally, obesity increases the mean disposition residence time of thiopental, and this is associated with a prolongation of terminal half‐life, although the increased adipose mass will serve to remove more drugs from the blood and speed clinical recovery from a single bolus [10]. Pharmacokinetic parameters of thiopental have been described in dogs, horses, sheep, and rabbits [11,12]. The initial volume of distribution was 38.1 ± 18.4 mL/kg in dogs, 44.5 ± 9.1 mL/kg in sheep, and 38.6 ± 10.0 mL/kg in rabbits [12]. Horses and ponies had large volumes of distribution: 783 ± 128 mL/kg and 1127 ± 86 mL/kg, respectively [11]. The elimination half‐life (t1/2) was shown to be shortest in the rabbit (43.1 ± 3.4 min) and longest in the sheep (251.9 ± 107.8 min) [12]. The t1/2 in the dog was 182.4 ± 57.9 min [12], and 147 ± 21 min in the horse [11,12]. A single dose of IV methohexital produces unconsciousness of short duration (8 ± 3 min in dogs). Recovery times compared to thiopental are faster with time to sternal recumbency and standing at 19 and 32 min, respectively [7]. Pharmacokinetic parameters in dogs receiving an IV dose of 10 mg/kg revealed both a rapid disposition phase (distribution half‐life = 0.87 min) and a rapid elimination phase (t1/2 = 24.1 min). The volume of distribution was 1602 ± 643 mL/kg while the total plasma clearance was 37.5 ± 8.07 mL/kg/min [7]. Administration of methohexital induces cardiopulmonary changes similar to thiopental. A recent study evaluating the effect of methohexital on laryngeal function dogs described adverse events after methohexital administration, including tachycardia, seizure‐like activity, vomiting, and regurgitation [13]. Mixed‐breed dogs receiving 15 mg/kg of thiamylal IV remained unresponsive to toe pinch for 17 ± 9 min, returned to sternal recumbency in 55 ± 31 min, and were standing after 93 ± 46 min. Cardiopulmonary values in these dogs were similar to dogs receiving thiopental. Pharmacokinetics reveal a rapid disposition phase (distribution half‐life = 38.9 min) and an extended elimination phase (t1/2 = 666 min). The volume of distribution was 3352 ± 1887 mL/kg while the total plasma clearance was 3.21 ± 1.27 mL/kg/min [7]. A recent study using thiamylal to induce and maintain anesthesia in calves for approximately 2 h reported mean recovery times of over 5 h [14]. A single IV dose of 20 mg/kg of pentobarbital in mixed‐breed dogs produces anesthesia of 64 ± 31 min. These dogs then needed 238 ± 52 min to return to sternal recumbency and 432 ± 22 min to stand [15]. Pentobarbital undergoes extensive hepatic metabolism and is totally dependent on the liver for biotransformation and elimination. Sheep and goats, however, are rapid metabolizers of the drug and require supplemental doses if anesthesia is to be maintained beyond 20–30 min. As noted previously, administration of barbiturates results in CNS depression and anesthesia. Following barbiturate administration, the electroencephalogram (EEG) is depressed in a dose‐dependent fashion. With the administration of thiopental, the awake α pattern progresses to δ and θ waves until there is burst suppression and a flat EEG [16]. The barbiturates appear to possess cerebroprotectant properties. Cerebral metabolism of oxygen (CMRO2) is reduced by up to 55% in a dose‐dependent fashion [17]. Cerebral blood flow and intracranial pressure (ICP) are also decreased in parallel with the reduction in CMRO2 [17]. Cerebral perfusion pressure, however, is usually not adversely affected because ICP decreases more than mean arterial pressure. Several studies have been performed evaluating thiopental as a cerebroprotectant and have shown that it may be of some clinical value. In dogs pretreated with thiopental and subjected to isolated brainstem ischemia, auditory evoked potentials were increased compared to those dogs that were not pretreated with thiopental [18]. Additionally, it was demonstrated that the mitigating effect of immediate post‐arrest hypothermia in dogs with postischemic encephalopathy might be enhanced by thiopental [19]. These properties make thiopental an appropriate choice for patients with intracranial disease or a history of seizures. Methohexital has been associated with CNS excitation and epileptiform seizures, making it a poor choice for patients with seizures. Intraocular pressure is reduced slightly by thiopental administration [20]. Administration of lower doses of thiopental results in a decrease in stroke volume and myocardial contractility [21]. A mild decrease in arterial blood pressure can be seen, but it is usually offset by a compensatory increase in heart rate. Venodilation after thiopental administration can lead to sequestration of red blood cells in the spleen, an increase in splenic size, and a decrease in packed cell volume. Vasodilation of cutaneous and skeletal blood vessels may also predispose the patient to hypothermia. Ventricular arrhythmias, particularly ventricular bigeminy, have also been demonstrated [22]. The incidence of these arrhythmias may be reduced with adequate ventilation and oxygenation prior to thiopental administration. Thiopental sensitizes the myocardium to epinephrine‐induced arrhythmias in many species studied [23]. Barbiturate administration for the induction of anesthesia causes dose‐dependent depression of ventilatory centers, decreasing the brain’s responsiveness to hypoxemia and hypercarbia [24]. There is a decrease in respiratory rate and minute ventilation [25]. Transient periods of apnea are commonly reported after large, rapidly administered doses of thiopental. Thiopental has also been shown to cause bronchoconstriction in the dog [26]. However, laryngeal reflexes may be less affected by thiopental administration compared to other induction agents; therefore, it may be a good choice for evaluation of laryngeal function [27]. Thiopental has also been shown to reduce mucociliary clearance in the dog [28]. Healthy patients have little change in hepatic or gastrointestinal function after induction of anesthesia with thiopental and only modest decreases in hepatic blood flow may be seen. Barbiturates stimulate an increase in microsomal enzymes, but only after 2 to 7 days of sustained drug administration [29]. While gastrointestinal effects such as diarrhea or intestinal stasis have not been reported with barbiturate administration, thiopental has been shown to decrease the tone of the lower esophageal sphincter in cats [30]. Renal blood flow may be decreased slightly by thiopental administration, most likely due to decreases in systemic blood pressure and cardiac output. A 15 mg/kg dose of thiopental in the dog resulted in a mean glomerular filtration rate of 2.04 ± 0.36 mL/min/kg, which did not differ significantly from other induction agents [31]. IV barbiturates easily cross the placenta and establish a dynamic equilibrium between maternal and fetal circulation. However, it should be remembered that the placental circulation passes through the liver before reaching the fetal CNS, thereby reducing overall drug exposure for most highly metabolized drugs. In a study performed on dogs, thiopental more profoundly depressed neurological reflexes in puppies born by cesarean section compared to propofol or epidural anesthesia [32]. Additionally, uterine blood flow transiently decreased in pregnant ewes induced with thiopental [33]. It should be noted that barbiturates do not produce antinociception (and analgesia only during unconsciousness); therefore, additional analgesics should be administered to patients undergoing painful procedures. In fact, at subanesthetic doses, the barbiturates may actually be hyperalgesic. However, this effect is controversial and is likely not clinically significant [34]. Documented breed‐associated differences in anesthetic pharmacokinetics and pharmacodynamics are uncommon. However, among canine breeds, Greyhounds are relatively deficient in the hepatic microsomal enzymes that are needed to metabolize the thiobarbiturates. This deficiency, along with lean bodies and low‐fat stores, potentially results in prolonged recoveries from thiopental anesthesia when larger doses are administered [29]. Barbiturate anesthetics are often not recommended for sighthound breeds (e.g., Greyhound, Irish Wolfhound, and Afghan Hound) but use of anesthetic‐sparing premedications and minimal thiobarbiturate doses has allowed safe anesthetic induction in these breeds with minimal delay in recovery. The pharmacokinetics of a single dose of thiopental at 11 mg/kg has been studied [11]. In that study, a three‐compartment open model best described the disposition kinetics of thiopental. In plasma, thiopental has a very rapid initial distribution phase (distribution half‐life = 1.4 ± 1.2 min and 1.3 ± 0.7 min in horses and ponies, respectively). While horses had a somewhat shorter t1/2 (147 ± 21 min) than ponies (222 ± 44 min), no obvious difference in clearance of the drug between horses (3.5 ± 0.5 mL/kg/min) and ponies (3.6 ± 0.8 mL/kg/min) was noted [11]. Thiopental should not be administered to horses without prior sedation with an a2‐adrenergic receptor agonist, as significant excitement and incoordination may result. Additionally, anesthesia induction with guaifenesin and thiopental in horses that have just undergone maximal exercise is not recommended [35]. The pharmacokinetics of thiopental in sheep has also been described using a three‐compartment open model [36]. The volume of distribution was 1005 ± 196 mL/kg, total body clearance was 3.5 ± 0.8 mL/kg/min, and t1/2 was 196 ± 64 min in that study. Time of awakening was 36.6 ± 6.36 min [36]. The authors suggest that the relatively short duration of action of thiopental should be attributed mainly to elimination of the drug by hepatic metabolism and uptake by body fat [36]. This theory differs from the widely held belief that redistribution of thiopental terminates its anesthetic action. Toutain et al. propose that this is perhaps due to differences in regional blood flow between sheep and monogastric species, although this remains unestablished [36]. Barbiturates are effective anesthetic agents in swine limited only by the difficulty of attaining IV access in these species [37,38], although care should be used when administering thiopental to hypovolemic pigs as the anesthetic requirement for thiopental is reduced by approximately 35% in these animals [39]. Barbiturates do not trigger malignant hyperthermia in swine. The induction doses of thiopental used in veterinary species are listed in Table 27.1. It should be noted that the dose required to induce anesthesia and facilitate endotracheal intubation is altered by premedications and the patient’s physical status. Patients that are premedicated with other CNS depressants, or those that are hypovolemic, hypoproteinemic, acidotic, and/or uremic, will require less thiopental to induce anesthesia. Thiopental should be administered IV, preferably through a catheter, and the dose should be titrated to effect. Induction of anesthesia is rapid, occurring in approximately 20–30 s after administration. Because periods of apnea are often reported after induction of anesthesia with thiopental, equipment to facilitate intubation with a cuffed endotracheal tube, and to assist ventilation should be immediately available. The duration of action of thiopental is quite short with the distribution/redistribution phase lasting 14.9 ± 3.3 min in dogs [6]. Table 27.1 Barbiturate dosages in various species. IV, intravenous. Perivascular administration of thiopental is associated with tissue necrosis, especially at higher concentrations (e.g., 5%). If inadvertent perivascular injection occurs, dilution of the drug should be attempted. This is best accomplished by injection of saline through the needle or catheter that still remains in place. At the same time, lidocaine can be injected to produce vasodilation and local anesthesia. In order to reduce the incidence of ventricular arrhythmias, IV lidocaine has been investigated as a co‐induction agent with thiobarbiturates. Thiopental administered IV to dogs at a dose of 11 mg/kg and lidocaine at a dose of 8.8 mg/kg produced a smooth induction with no arrhythmias and less cardiovascular depression as compared to thiopental alone [40]. This technique may not be suitable for all species since the IV administration of a relatively large dose of lidocaine may cause toxicity. Mixtures of thiopental and propofol (1:1) have also been used in the dog [41]. The dose of each drug required for induction was reduced and induction quality was similar to that of thiopental or propofol alone. Recovery times and quality of recovery were similar to those of propofol and superior to those of thiopental alone [41]. It should be noted that the mixture should inhibit bacterial growth and is bactericidal after 48 h against Staphylococcus aureus, Escherichia coli, Pseudomonas aeruginosa, and Candida albicans [42]. Mixtures of thiopental and propofol at a ratio of less than 1:1 do not maintain bactericidal properties [42]. Proper evaluation of drug stability in these mixtures is lacking, but they appear to maintain relatively normal potency. Propofol is chemically distinct from all other IV drugs used to induce or maintain anesthesia. Awakening from anesthesia has been found to be more rapid and complete with propofol than with other induction agents. It is this rapid return to consciousness with minimal residual effects that has made propofol such a popular anesthetic induction agent in both human and veterinary anesthesia. Its first use was reported in 1977 [43]. Propofol is a substituted isopropylphenol (2,6‐diisopropylphenol) that can be used to produce sedation, as well as to induce and maintain anesthesia (Fig. 27.2A). It is formulated as proprietary variations of an oil‐in‐water emulsion containing 1% propofol, 10% soybean oil, 2.25% glycerol, and 1.2% purified egg phosphatide. Propofol is relatively insoluble in aqueous solutions but is highly lipid soluble. It is a slightly viscous, milky white substance with a pH of 6.5–8.5. This formulation of propofol is stable at room temperature and is not light‐sensitive. Most formulations do not contain preservatives and therefore will support bacterial or fungal growth; therefore, strict aseptic technique should be used when using multidose vials of propofol [44]. According to most labels, opened vials should be used or discarded before 6 h. When using propofol as a constant rate infusion, it would be prudent to discard IV tubing and administration sets every 12 h or if gross bacterial contamination occurs. Figure 27.2 Chemical structure of A. propofol and B. fospropofol. Source: National Center for Biotechnology Information [50]. A lipid‐free microemulsion formulation of propofol has been developed to increase vial shelf‐life when broached, reduce infection risk by the addition of antimicrobial agents, reduce inherent emulsion instability, and reduce pain on injection [45,46]. It has a shelf‐life of 28 days once the vial is opened. Additionally, a propofol formulation for the veterinary market containing benzyl alcohol has also been developed for use in dogs which has an increased shelf‐life of 28 days once the vial is opened [47]. Like barbiturates, propofol appears to exert its anesthetic effects through an interaction with GABAA receptors [48]. Propofol also inhibits the N‐methyl‐D‐aspartate (NMDA) receptor through modulation of channel gating which may also contribute to its CNS effects [49]. Propofol pharmacokinetics can be described by a two‐compartment open model, with a rapid distribution phase followed by a slower clearance phase [51]. Following IV injection of propofol, it rapidly moves into the CNS resulting in induction of anesthesia. It is then rapidly redistributed from the brain to other tissues in the body, terminating its anesthetic action. In most species (cats are an exception), propofol undergoes rapid and extensive hepatic metabolism resulting in the production of inactive water‐soluble sulfide and glucuronide metabolites. These are then excreted by the kidneys [52]. Clearance of propofol from the plasma exceeds hepatic blood flow, which emphasizes the importance of tissue uptake and suggests that extrahepatic metabolism or extrarenal excretion may occur [53]. In humans, there is no evidence of impaired elimination of propofol in patients with cirrhosis of the liver and extrahepatic metabolism has been confirmed in the anhepatic phase of liver transplant patients [53,54]. In cats, extrahepatic metabolism of propofol has been demonstrated in pulmonary tissue [55]. In cats with hepatic lipidosis, anesthetic induction with propofol for placement of a feeding tube did not increase morbidity or mortality despite the anticipated alteration of drug action [56]. The pharmacokinetics of propofol have been thoroughly studied in the dog [51,57,58]. Propofol’s lipophilic nature results in a large apparent volume of distribution (17.9 L/kg) as well as steady‐state volume of distribution (9.7 mL/kg) [51]. Due to the rapid redistribution of the drug into other tissues as well as rapid and extensive metabolism, the initial distribution half‐life is short as is the rate of disappearance from plasma. Greyhounds appear to have a smaller apparent volume of distribution and steady‐state volume of distribution, which suggests recovery from propofol anesthesia in Greyhounds will be slower [58]. Dogs greater than 8.5 years of age also have been shown to have a slower clearance rate compared to younger dogs [57]. The rapid distribution and clearance of propofol make it a suitable choice for maintenance of anesthesia by constant rate infusion of the drug in many species. In most species (except cats), propofol does not accumulate after repeated doses and/or prolonged infusion of the drug, so recovery from anesthesia remains rapid and of good quality [59,60]. IV injection of propofol results in rapid CNS depression and induction of anesthesia. Much like the barbiturates, propofol reduces ICP and CMRO2 [61]. Cerebral perfusion pressure is reduced slightly in patients with normal ICP, but in patients with elevated ICP, the drop in cerebral perfusion pressure is significant and may not be beneficial [62]. Brain responsiveness to carbon dioxide and cerebral metabolic autoregulation is maintained during propofol administration [61]; however, this response may be modified by concurrent administration of other drugs such as opioids. Propofol produces cortical EEG changes similar to barbiturates, including the incidence of burst suppression with the administration of high doses [61]. Propofol possesses anticonvulsant effects and can be used in the treatment of seizures [63,64]. The most prominent cardiovascular effect of propofol administration is a decrease in arterial blood pressure. Decreases in systemic vascular resistance and cardiac output are also seen [65,66]. The magnitude of myocardial depression and vasodilation appear to be dependent on dose (and plasma concentration) [65]. These cardiovascular effects may be more profound in patients that are hypovolemic [67], geriatric, or have compromised left ventricular function [68]. Unlike thiopental, however, administration of propofol does not usually result in a compensatory increase in heart rate. Propofol does appear to sensitize the myocardium to epinephrine‐induced arrhythmias but does not appear to be arrhythmogenic [69]. Much like thiopental, propofol causes dose‐dependent depression of ventilation and postinduction apnea (PIA), with transient cyanosis occurring regularly [70]. The incidence of apnea appears to be related to dose and rate of administration, with rapid injection rates making apnea more likely to occur [70]. The ventilatory response to hypoxemia [71] and carbon dioxide [72] is reduced by propofol [73], and the administration of opioids may enhance propofol’s effect on ventilation [74]. Respiratory effects are also seen when propofol is administered as a constant rate infusion, with propofol decreasing tidal volume and respiratory rate [72]. Propofol does not adversely affect hepatic blood flow [75] or glomerular filtration rate in dogs [31]. In humans, propofol has been shown to be a very effective antiemetic. In fact, subanesthetic doses of propofol may be used postanesthesia to treat nausea and vomiting [76]; however, this effect has not been demonstrated in domestic animals. Like thiopental, propofol produces excellent muscle relaxation. Occasionally, however, myoclonic movements have been reported in both humans and dogs [77,78]. These movements resolve spontaneously. Propofol readily crosses the placenta, but it is rapidly cleared from the neonatal circulation [79]. It is considered an acceptable choice for dogs undergoing cesarean section, as effects on healthy puppies are minimal [80]. Propofol produces neither antinociception nor hyperalgesia [34]. Animals undergoing painful procedures should therefore receive appropriate analgesics as part of the anesthetic plan. Propofol can be administered IV for sedation and induction of anesthesia. It is also well suited to be used as a constant rate infusion for the maintenance of anesthesia. Propofol is often recommended for use in Greyhounds. The dose required for induction of anesthesia in Greyhounds is the same as mixed‐breed dogs, but the recovery time is longer in Greyhounds [52]. As mentioned previously, the lipid‐based emulsion formulation of propofol is capable of supporting microbial growth. In a retrospective study of clean wounds in dogs and cats, animals receiving propofol were 3.8 times more likely to develop wound infections compared to animals that did not receive propofol [81]. Causal mechanisms have not been established and the numerous confounding factors of wound infection rates would caution against overemphasizing the results of this study. However, strict aseptic technique should be followed when using propofol as an anesthetic and prompt disposal of unused drug should reduce the potential for drug contamination and any impact that would have on infection rates. The lipid‐free microemulsion formulation of propofol has been evaluated in the dog [82] and appears to be pharmacokinetically and pharmacodynamically similar to the lipid formulation. However, it has been reported that IV injection of the microemulsion formulation resulted in severe pain and complications in dogs [83]. Repeated daily administration of propofol can induce oxidative injuries to feline red blood cells [84]. Heinz body formation, facial edema, generalized malaise, anorexia, and diarrhea were all reported in one study in which cats received propofol on consecutive days. Heinz body formation was significantly increased by the third day of propofol administration and recovery times were significantly increased after the second consecutive day [84]. However, in another study, repeated propofol anesthesia was evaluated and no relevant hematologic changes were reported [85]. The formulation of propofol containing benzyl alcohol (PropofloTM28) has been evaluated in cats [47]. While there has been concern regarding this formulation due to potential adverse effects of benzyl alcohol on feline blood and nervous systems, it has been shown that administration to healthy cats of normal to high clinical doses of the formulation did not cause organ toxicity [47]. The lipid‐free microemulsion formulation has also been evaluated in cats [46,86] and, when compared to the lipid‐emulsion formulation, produces comparable pharmacokinetic, pharmacodynamic, and physiologic responses [46]. The clinical effects of propofol in the horse are similar to those seen in other species. IV administration of propofol following sedation produces rapid induction of anesthesia, a short duration of action, and a rapid, smooth recovery [87,88]. However, propofol can produce unpredictable behavioral responses and excitation at the time of induction, thereby limiting its use as a routine induction agent in adult horses [89]. These adverse effects at the time of induction appear to be prevented; however, by the IV administration of guaifenesin for 3 min prior to administration of propofol [90]. Propofol, either solely or in combination with other drugs, has been used effectively as a constant rate infusion in horses to maintain anesthesia [91–93]. While adverse events at induction were noted with propofol administration, horses appeared calm and coordinated in recovery [89]. This prompted the investigation of propofol, in combination with xylazine, as a potential modulator of recovery [94,95]. In these reports, it was noted that a combination of xylazine and propofol might be of some benefit as the quality of recovery was significantly improved [94,95]. The lipid‐free microemulsion formulation of propofol has also been evaluated in the horse [96,97]. A 3‐h continuous rate infusion produced similar cardiopulmonary and biochemical results to those reported when the lipid formulation was used [97]. However, in a different study of the pharmacokinetics, investigators urged caution when using propofol to maintain anesthesia due to variable kinetics, poor analgesia, and myoclonic activity [96]. Propofol has been satisfactorily used as an induction agent in sheep [98], goats [99], cows [100], and camelids [101]. Reported characteristics of propofol anesthesia in these species are similar to those reported in other veterinary species. Induction is rapid and smooth, duration of action is short, and recovery is of good quality. Cardiopulmonary effects are similar to those reported in other species. The pharmacokinetics of propofol have been described in the goat. The mean t1/2 was short (15.5 min), the volume of distribution at steady state was large (2.56 L/kg), and the clearance rate was rapid (275 mL/kg/min) [99]. These values differ from those obtained in sheep where the mean t1/2 was 56.6 ± 13.1 min, volume of distribution was 1.037 ± 0.48 L/kg, and clearance was 85.4 ± 28.0 mL/kg/min [102]. Propofol does not induce malignant hyperthermia in susceptible swine [103]. As with other species, respiratory depression and apnea have been reported with propofol administration [104]. Propofol doses commonly used in veterinary patients are given in Table 27.2. It should be noted that the dose required to achieve endotracheal intubation is altered by premedications and the physical status of the patient. Patients that are debilitated and/or have received CNS depressants will often require less propofol for induction. Propofol should be administered IV, preferably through a catheter, and the dose should be titrated to effect. Once administered, induction of anesthesia with propofol occurs within 20–30 s, although in states of low cardiac output, the lag period may be prolonged. The quality of induction with propofol is good with a smooth transition to unconsciousness. To reduce the incidence of PIA, it is often recommended that the titrated dose of propofol should be administered slowly (over 60–90 s); however, apnea may still occur. Transient apnea is seldom a problem (except when establishment of a patent airway is difficult or impossible such as with some oropharyngeal conditions) as long as the anesthetist is prepared to intubate with an endotracheal tube and assist or control ventilation until spontaneous respiration begins. Duration of unconsciousness ranges from 2–8 min and the quality of recovery is good. While perivascular injection of propofol will not produce anesthesia, it is not associated with tissue necrosis. Pain on IV injection has been reported and appears to be associated with injection of propofol into smaller vessels, not necessarily perivascular injection. To decrease the incidence of injection pain, clinicians may choose to inject propofol into larger vessels, administer a small dose of lidocaine IV prior to propofol injection, or dilute the dose by administering propofol into a running IV fluid line. As stated previously, propofol is relatively insoluble in aqueous solutions and is therefore formulated as an oil‐in‐water emulsion. Disadvantages of this type of formulation have been discussed previously (e.g., pain at the injection site and potential bacterial contamination). Other drawbacks of the emulsion formulation include propofol infusion syndrome and hypertriglyceridemia with prolonged infusion. Research has therefore focused on finding alternative formulations or related drugs to address these issues. Fospropofol is a water‐soluble prodrug of propofol licensed by the United States Food and Drug Administration [107]. It is chemically described as a 2,6‐diisopropylphenoxymethyl phosphate disodium salt (Fig. 27.2B). Following administration, it is metabolized by alkaline phosphatase producing propofol, phosphate, and formaldehyde. The formaldehyde is rapidly metabolized by aldehyde dehydrogenase in the liver and erythrocytes into formate. Systemic formaldehyde levels do not increase beyond those occurring from food and environmental intake or metabolism [107]. The available formulation of fospropofol is an aqueous, colorless, and clear solution supplied in a single dose vial at a concentration of 35 mg/mL. The pharmacokinetics are complex and reliable data on the kinetics are lacking as most publications on the subject have been retracted due to potential analytical errors [108]. In dogs, a single IV dose of 6 mg/kg of fospropofol resulted in a significantly slower onset (124 ± 15 s) and longer duration (1448 ± 155 s) of unconsciousness than propofol. This is unsurprising as the prodrug requires enzymatic conversion to the active drug [45]. In rabbits receiving infusions of fospropofol, the minimum infusion rate (MIR) was 2.0 mg/kg/min compared to 0.9 mg/kg/min for propofol and recovery time increased as the duration of infusion increased [109]. Rabbits receiving infusions of fospropofol for 6 or 8 h needed significantly more time (52 ± 6 and 84 ± 10 min, respectively) to recover than those receiving propofol (36 ± 7 and 48 ± 5 min, respectively) [109]. Table 27.2 Propofol dosages in various species. a Premedicated b Martin‐Cancho et al. [105]; Terada et al. [106]. IV, intravenous. Additional water‐soluble prodrugs of propofol have shown promise and are under investigation. HX0507 was found to have anesthetic effects similar to propofol and, in dogs, was found to cause similar dose‐dependent hypotension but less dose‐dependent respiratory depression [110]. Also, in development is HX0969w, a water‐soluble ester prodrug of propofol that is similar to fospropofol, but releases γ‐hydroxybutyrate instead of formaldehyde [111]. With modifications to the HX0969w molecule, studies in rats show greater water solubility, faster onset, and similar anesthetic effects as fospropofol [112]. While these new formulations are promising, they must offer significant advantages over the standard propofol emulsion to justify their incorporation into clinical practice. The dissociatives are phencyclidine derivatives that produce a so‐called state of “dissociative anesthesia” characterized by dissociation of the thalamocortical and limbic systems causing a change in awareness [113]. Ketamine hydrochloride and tiletamine hydrochloride are the dissociative anesthetics most commonly used in veterinary medicine. Ketamine is 2‐(o‐chlorophenol)‐2‐(methylamino)‐cyclohexanone hydrochloride (Fig. 27.3A). Two optical isomers of ketamine exist due to an asymmetric carbon. Most formulations contain the racemic mixture, but a purified S‐ketamine formulation is available in some countries. The S(+)‐enantiomer produces more intense analgesia, is metabolized more rapidly, and has a lower incidence of emergence reactions than the R(–)‐enantiomer [114]. Racemic ketamine is available as a 10% aqueous solution. It has a pH of 3.5–5.5 and is preserved by benzethonium chloride. Tietamine is 2‐(ethylamino)‐2‐(2‐thienyl)‐cyclohexanone hydrochloride (Fig. 27.3B). It is only available in combination with the benzodiazepine, zolazepam, and is marketed under the names Telazol® and Zoletil®. Tiletamine–zolazepam combinations are available as a white powder that is reconstituted with 5 mL of diluent. The final concentrations of tiletamine and zolazepam depend on the product being used. Figure 27.3 Chemical structure of A. ketamine and B. tiletamine. Source: National Center for Biotechnology Information [115]. The dissociative anesthetics act on NMDA, opioid, monoaminergic, and muscarinic receptors. Additionally, they interact with voltage‐gated calcium channels [116,117]. Interestingly, the dissociatives do not appear to interact with GABA receptors as the other injectable anesthetics do. Ketamine and tiletamine are non‐competitive antagonists at the NMDA receptor. They bind to the phencyclidine‐binding site, which prevents glutamate, an excitatory neurotransmitter, from binding. Prevention of glutamate binding results in depression of the thalamocortical, limbic, and reticular activating systems. Dissociatives have also been reported to have action at μ‐, δ‐, and κ‐opioid receptors [118]. Activity at the opioid receptors imparts analgesic properties unlike other injectable anesthetics, although the clinical significance of this action at clinically relevant doses is debatable. Additionally, the dissociatives’ interaction at monoaminergic receptors may also contribute to antinociception [117]. Because dissociative anesthesia is associated with anticholinergic symptoms (emergence delirium, bronchodilation, and sympathomimetic actions), it is thought that these drugs have antagonist activity at the muscarinic receptors [117]. However, many of these effects may also be related to the sympathetic nervous system‐stimulating effects of ketamine and tiletamine. The dissociatives are similar to other injectable anesthetics in that they have a rapid onset of action (especially when given IV), short duration, and are highly lipophilic. Unlike other injectable anesthetics, the dissociatives are effective when administered IM. Peak plasma concentrations occur within 1 min of IV administration and 10 min following IM injection. The high lipid solubility of the dissociatives ensures that the blood–brain barrier is crossed quickly, which establishes effective brain concentrations of the drugs [119]. In most species, metabolism of the dissociatives occurs in the liver. Ketamine is demethylated by hepatic microsomal enzymes producing the active metabolite norketamine. Eventually, norketamine is hydroxylated and then conjugated to form water‐soluble and inactive glucuronide metabolites that are excreted by the kidney [120]. This process differs in the cat, where ketamine is biotransformed to norketamine, which is excreted unchanged in the urine [121]. Dissociatives should be used with care in animals with significant hepatic and/or renal dysfunction as prolonged anesthetic times may result. Tiletamine also undergoes hepatic metabolism and renal excretion. Since tiletamine is only supplied with zolazepam, the action of the benzodiazepine should also be discussed. In cats, the duration of action of zolazepam is longer than that of tiletamine. This means that the CNS effects of the benzodiazepine (sedation) are present longer than those of tiletamine. In the dog, the reverse is true; the duration of action of tiletamine is longer than zolazepam. This means that the effects of the dissociative are observed, including muscle rigidity, sympathetic stimulation, and emergence delirium. Pigs appear to have a slow, calm recovery from tiletamine–zolazepam combinations, while in horses an agitated recovery may be seen if additional sedation is not provided. If significant plasma levels of tiletamine are present, reversal of the benzodiazepine with flumazenil may result in an anxious recovery. Dissociative anesthesia resembles a cataleptic state in which the patient does not appear to be asleep but does not respond to external stimuli. As mentioned previously, antagonism of the NMDA receptor leads to a dissociation of the limbic and thalamocortical systems [122]. Unlike other injectable anesthetics, the dissociatives increase cerebral blood flow and CMRO2 [123]. Cerebral vasodilation and an increase in blood pressure results in an increase in ICP [122]. It appears that this increase in ICP can be attenuated if ventilation is controlled and animals remain eucapnic [124]. Administration of thiopental or a benzodiazepine has also been shown to reduce ketamine‐induced increases in ICP [119]. Clinicians should still exercise caution, however, when using dissociatives in patients that have or are suspected of having increased ICP. Eleptiform EEG patterns are seen after ketamine administration [125], resulting in the recommendation that dissociatives should be avoided in patients with seizure disorders. However, it has been shown that ketamine does not alter the seizure threshold in epileptic patients [126]. Additionally, there is evidence that ketamine possesses anticonvulsant as well as neuroprotective activity [127]. Abnormal behavior that may progress to emergence delirium may occur during recovery from dissociative anesthesia. Misrepresentation of visual and auditory stimuli may be responsible for this reaction [120]. A patient experiencing an emergence reaction may be ataxic, hyperreflexic, sensitive to touch, have increased motor activity, and may have a violent recovery. These reactions are usually temporary and usually resolve within a few hours. Administration of CNS depressants such as benzodiazepines, acepromazine, or α2‐adrenergic receptor agonists may decrease the incidence and/or the severity of these reactions [120]. Ketamine has a direct negative cardiac inotropic effect [128], but it is usually overcome by central sympathetic stimulation. IV administration of ketamine increases systemic and pulmonary arterial pressures, heart rate, cardiac output, myocardial oxygen requirements, and cardiac work [129]. It is likely that these changes are the result of direct stimulation of the CNS leading to increased sympathetic nervous system outflow [130]. Ketamine also inhibits norepinephrine reuptake into postganglionic sympathetic nerve endings leading to an increased concentration of plasma catecholamines [131]. Critically ill patients may respond to induction of anesthesia with ketamine with a decrease in systemic blood pressure and cardiac output. The catecholamine stores and the sympathetic nervous system’s compensatory mechanism may be exhausted, unveiling ketamine’s negative inotropic effects [132]. While healthy animals are usually tolerant of increased cardiac work, myocardial oxygen requirements, and heart rate, ketamine should be used with caution in those animals that have severe cardiovascular disease (e.g., uncontrolled hypertension, cardiomyopathy, or heart failure), and/or are already tachycardic or dysrhythmic. Stimulation of the cardiovascular system in these patients may not be desirable. Unlike other injectable anesthetics, ketamine does not cause significant respiratory depression. Ventilatory responses to hypoxia and carbon dioxide are maintained in animals receiving ketamine as the sole anesthetic agent [133]. When ketamine is administered with other CNS depressants, significant respiratory depression can occur. Ketamine administration has been associated with an “apneustic” respiratory pattern, characterized by a prolonged inspiratory duration and relatively short expiratory time [134]. Despite this altered respiratory pattern, carbon dioxide levels and minute ventilation usually remain within normal limits. Ketamine is a bronchial smooth muscle relaxant and causes bronchodilation and a decrease in airway resistance [135]. This makes it an attractive choice when anesthetizing animals with asthma or obstructive airway diseases such as chronic obstructive pulmonary disease. Pharyngeal and laryngeal reflexes remain intact when ketamine is used as the sole anesthetic agent [136]. It should be noted, however, that these reflexes are often uncoordinated and not protective. An endotracheal tube should always be placed to prevent aspiration. Maintaining a secure airway is especially important because ketamine increases salivation and respiratory tract secretions [119]. These can be reduced with the administration of an anticholinergic. Laboratory tests that indicate hepatic or renal function are not altered by the administration of dissociatives. Gastrointestinal motility is unchanged after the administration of ketamine in the dog [137]. As previously discussed, the action of the dissociatives at NMDA and opioid receptors imparts analgesic properties. In fact, it has been demonstrated that subanesthetic doses of ketamine produce profound analgesia, especially in situations of somatic pain [116]. Additionally, blockade of the excitatory neurotransmitter glutamate at the NMDA receptor by the dissociatives is thought to play a role in preventing or minimizing central sensitization or wind‐up pain. Therefore, preemptive administration of ketamine prior to painful stimuli may play a role in attenuating central sensitization [138,139]. Unlike the previously discussed injectable anesthetic agents, the dissociatives produce little muscle relaxation. In fact, the dissociatives may cause muscle rigidity and often spontaneous movement of the limbs, trunk, and/or head. Substantial increases in intraocular pressure (IOP) are seen after ketamine administration which may be a result of increased tone of the extraocular muscles [140]. Muscle relaxation can be improved with the co‐administration of benzodiazepines or α2‐adrenergic receptor agonists. Ketamine crosses the placenta and enters fetal circulation. In a study evaluating neurologic reflexes in puppies born via cesarean section, anesthetic induction of the dam with ketamine and midazolam resulted in the most depression of neurologic reflexes when compared with other injectable induction drugs [32]. The dissociatives can be administered either IV or IM to produce a range of effects from sedation to anesthesia. Induction of anesthesia with ketamine alone can lead to muscle rigidity, spontaneous movement, and undesirable recoveries. Therefore, it is usually administered with a co‐induction agent such as a benzodiazepine. Because tiletamine is supplied as a combination with zolazepam, there is no need for additional benzodiazepine for IV administration. IM ketamine or tiletamine–zolazepam combinations are frequently combined with an α2‐adrenergic receptor agonist and an opioid to produce excellent immobilization with muscle relaxation and analgesia. Table 27.3 shows dosages of ketamine alone or in combination with other anesthetics commonly used in the dog. Table 27.4 shows common doses and usage of Telazol® in dogs. The dissociatives have been used to produce a range of effects from sedation to anesthesia in cats. These drugs can be administered IV or IM. Ketamine is also absorbed through the oral mucosa. In particularly fractious cats, ketamine can be sprayed into the mouth to effectively induce sedation and facilitate the induction of anesthesia [141]. Copious salivation usually results due to the bitter taste and/or low pH of ketamine. α2‐Adrenergic receptor agonists, benzodiazepines, and/or acepromazine are commonly administered in combination with IM ketamine. The combination of dexmedetomidine, ketamine, and an opioid produces excellent chemical restraint/anesthesia, muscle relaxation, and analgesia [142]. Tiletamine–zolazepam combinations (500 mg) have been reconstituted with xylazine (100 mg) and ketamine (400 mg) to form a potent chemical restraint/anesthesia cocktail that can be administered IM [143,144]. Care must be exercised when administering the drug combination as the overall volume is very small and accurate measurement is important. Additionally, careful patient monitoring to prevent profound hypothermia is required since prolonged recovery may result due to a reduction in metabolic rate. Flumazenil and/or an α2‐adrenergic receptor antagonist has been used to speed recovery from this combination. Table 27.5 shows common combinations and dosages of Telazol® in cats. The use of tiletamine–zolazepam combinations in large felids, especially tigers, is not recommended. Adverse reactions, including delayed recovery, hindlimb paresis, hyperreflexia, seizures, and death have been reported [145]. Table 27.3 Commonly used ketamine dosages alone or in combination in dogs. IM, intramuscular; IV, intravenous. Table 27.4 Commonly used tiletamine–zolazepam (Telazol®) dosages alone or in combination in dogs. IM, intramuscular; IV, intravenous. Table 27.5 Commonly used tiletamine–zolazepam (Telazol®) dosages alone or in combination in cats. IM, intramuscular; IV, intravenous. The dissociatives, particularly ketamine, are used extensively in equine anesthesia. IV administration of the dissociatives rapidly and smoothly induces anesthesia provided that adequate sedation has been achieved prior to their administration. If the dissociatives are administered before adequate sedation has been provided, excitement will occur. Some muscle rigidity and involuntary movement may still occur when ketamine is used; therefore, it is frequently combined with a co‐induction agent such as a benzodiazepine, α2‐adrenergic receptor agonist, or guaifenesin. Anesthesia can be maintained by administering additional IV doses of ketamine. These doses may be administered as intermittent boluses or as a constant rate infusion. When used as a constant rate infusion, ketamine is frequently combined with sedatives and analgesic agents (e.g., α2‐adrenergic receptor agonists, guaifenesin, and opioids) in a combination commonly referred to as “triple drip.” Commonly used doses for ketamine in combination with other anesthetic agents can be found in Table 27.6. Tiletamine–zolazepam combinations can be administered IV to induce anesthesia in the horse after adequate sedation has been achieved. It should be noted, however, that recovery from tiletamine–zolazepam anesthesia is sometimes associated with excitement and incoordination if additional sedatives such as α2‐adrenergic receptor agonists are not administered, or anesthesia is not prolonged with inhalants [151]. Table 27.7 shows dosages for tiletamine in horses. The dissociatives can be used in ruminants to induce anesthesia. Sedation and muscle relaxation are usually improved by the administration of an α2‐adrenergic receptor agonist or benzodiazepine prior to the administration of ketamine. Anesthesia can be maintained with a constant rate infusion of ketamine or by a combination of ketamine, guaifenesin, and xylazine. Subanesthetic doses of ketamine (in combination with xylazine) have been administered to calves to produce sedation prior to castration [152]. This so‐called “ketamine stun” technique may be an efficacious and cost‐effective alternative or adjunct to local anesthesia for castration [152]. See Table 27.7 for dosages of the dissociatives in ruminants. The dissociatives have been used extensively in swine for chemical restraint and anesthesia. Ketamine does not induce malignant hyperthermia in susceptible pigs, although its use in these animals has been controversial [153]. Similar to other species, ketamine as a sole anesthetic agent produces poor muscle relaxation so it is commonly combined with azaperone, benzodiazepines, and/or α2
27
Injectable Anesthetics
Introduction
Barbiturates
Chemical structure
Mechanism of action
Pharmacokinetics
Thiopental
Methohexital
Thiamylal
Pentobarbital
Pharmacodynamics
Central nervous system
Cardiovascular system
Respiratory system
Hepatic, renal, and gastrointestinal systems
Fetal/neonatal effects
Analgesic effects
Species‐specific effects
Canine
Equine
Ruminant
Swine
Clinical use
Thiopental mg/kg IV
Pentobarbital mg/kg IV
Dog
8–22
2–30
Cat
8–22
2–15
Horse
4–15
Cow
4–22
Sheep
8–15
20–30
Llama
6–15
Pig
5–12
Propofol
Chemical structure
Mechanism of action
Pharmacokinetics
Pharmacodynamics
Central nervous system
Cardiovascular system
Respiratory system
Hepatic, renal, and gastrointestinal systems
Muscle
Fetal/neonatal effects
Analgesic effects
Species‐specific effects
Canine
Feline
Equine
Ruminant
Swine
Clinical use
Fospropofol and other propofol prodrugs
Induction dose (mg/kg) IV
Constant rate infusion (mg/kg/min)
Dog
3a–10
0.2–0.6
Cat
5–10
0.2–1.0
Horse
2–3a, 6–8
0.2–0.4
Foal
2
0.33
Donkey
2
0.21
Pig
2–3
0.1–0.2
Llama
2
0.4
Ferret
2–4
Rabbit
2–10
0.6–0.9b
Sheep
2–6
0.3–0.4
Goat
3–6
0.3
Dissociatives
Chemical structure
Mechanism of action
Pharmacokinetics
Pharmacodynamics
Central nervous system
Cardiovascular system
Respiratory system
Hepatic, renal, and gastrointestinal systems
Analgesic effects
Muscle
Fetal/neonatal effects
Species‐specific effects
Canine
Feline
Agent
Dose (mg/kg)
Route
Duration (min)
Effect
Ketamine alone
10
IV
7–23
Short duration, anesthesia inadequate for surgery
Acepromazine
Ketamine
0.2
10
IV
31–47
Clinical anesthesia, less muscle rigidity
Acepromazine
Ketamine
0.22
11–18
IM
Restraint, spastic movements, prolonged recovery
Xylazine
Ketamine
0.55–1.1 IM
22 IV to effect
IM/IV
28–36
Surgical anesthesia, muscle relaxation, analgesia for abdominal surgery
Atropine
Xylazine
Ketamine
0.04
1.1
11
IV
17–35
Increased risk with dogs with cardiopulmonary compromise
Atropine
Xylazine
Ketamine
0.04
1.1
22
IM
17–35
Increased risk with dogs with cardiopulmonary compromise
Medetomidine
Ketamine
0.04
5
IM
25–35
Longer muscle relaxation and recovery than xylazine/ketamine
Diazepam
Ketamine
0.28
5.5
IV
Suitable induction for sighthounds
Midazolam
Ketamine
0.5
10
IV
10–16
Increased heart rate, mild respiratory depression, good muscle relaxation
Agent
Dose (mg/kg)
Route
Duration (min)
Effect
Telazol®
9.9
IM
10–30
Rough recovery
Telazol®
6.6
IV
7–27
Telazol®
Xylazine
Butorphanol
8.8
1.1
0.22
IM
100
Clinical anesthesia, good muscle relaxation, good analgesia
Butorphanol
Telazol®
0.7
9.3–11.9
IM
Unsatisfactory sedation in vicious dogs
Acepromazine
Telazol®
0.6–3.0
17.5–21.1
IM
Adequate sedation in vicious dogs
Agent
Dose (mg/kg)
Route
Duration (min)
Effect
Telazol® alone
6–40 (average 12.8)
IM
40–70
Salivation, apneustic breathing
Telazol® alone
12.8
IV
35–70
Salivation, apneustic breathing
AcepromazineTelazol®
0.13.4 ± 1.09
IM
Adequate anesthesia for castration
Telazol®KetamineXylazine
3.32.640.66
IM
34–52
Smooth induction and recovery, excellent muscle relaxation, good analgesia
Equine
Ruminant
Swine
Stay updated, free articles. Join our Telegram channel
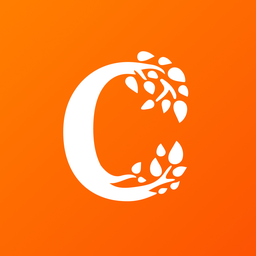
Full access? Get Clinical Tree
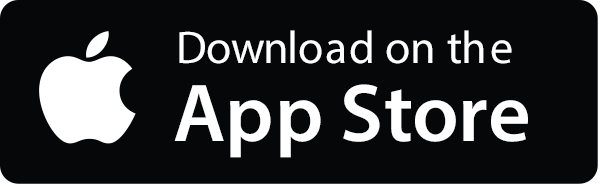
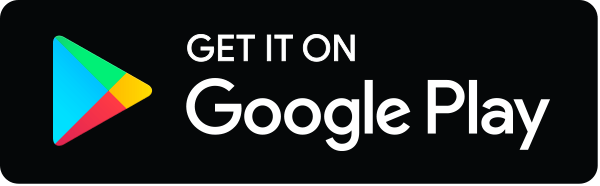