Bone is a specialized form of connective tissue that functions as an integral part of the locomotor system. Bones act as lever arms during motion, provide resistance to the effects of gravitational force on the body, and provide protection and support to adjacent structures. Bone also serves as a reservoir of mineral for systemic mineral homeostasis.1,2 Bones differ in shape and function and include long, flat, intramembranous, woven, and compact bones. Specialized connective tissue called periosteum surrounds the outside surface of the bones and provides protection and nutrition. Long bones are divided into epiphyseal, physeal, metaphyseal, and diaphyseal regions. Woven, cancellous, and lamellar bone is found adjacent to the physis in the epiphyseal and metaphyseal regions; compact or cortical bone surrounds the marrow cavity in the diaphyseal region.1,2 The regulation of mineral ions in the serum is controlled mainly by parathyroid hormone, calcitonin, and vitamin D. Parathyroid hormone increases resorption of bone by stimulating osteoclastic activity to increase serum calcium. Calcitonin inhibits the activity of osteoclasts and inhibits intestinal and renal calcium absorption. Vitamin D acts on the intestine to increase absorption of calcium and phosphorous and directly on bone by both mobilization of calcium and phosphorous from previously formed bone and promotion of maturation and mineralization of bone matrix. Bone is an active living tissue that is able to adapt and react, although somewhat slowly, to forces applied to the musculoskeletal system. Although bone can react to external stimuli or forces, it has limited methods of reaction: bone production, bone resorption, or a combination of production and resorption.1–3 Bone is formed and grows by means of intramembranous ossification, endochondral ossification, or both. Intramembranous bone formation begins with proliferation of mesenchymal cells that transform into osteoblasts that form matrix, which is then calcified. A fibrovascular layer develops on the internal and external surfaces of the bone to provide nutrition and osteogenic cells to allow the continued production and resorption of the bone. Intramembranous bone formation occurs primarily in the bones of the calvarium and mandible. The bones of the extremities, spine, and pelvis form by both intramembranous and endochondral ossification, with endochondral ossification predominant in the long bones. Endochondral (intracartilaginous) ossification progresses by the formation of a cartilage model derived from the mesenchyma that is then replaced with bone. Long (tubular) bones begin by a primary center of ossification in the center of the cartilaginous model and grow with intramembranous ossification, with secondary centers appearing later at the ends within the epiphyses and apophyses that continue the growth by endochondral ossification. The physis is organized into five clearly demarcated histologic zones: (1) resting zone containing immature cells on the epiphyseal side of the physis, (2) cell growth or proliferation, (3) cell hypertrophy, (4) provisional calcification, and (5) ossification. The weakest zone is in the area of the hypertrophied cartilage cells; this is the most common area of physeal fracture following trauma.1–3 Bone healing is similar to the endochondral growth process.2,3 Bone healing is a normal ongoing process throughout all bones as a result of aging of the bone that requires replacement of bone over time and healing of microfractures, which if not repaired could result in structural failure of the bone and clinical fracture. Processes that interfere with normal bone metabolism or physical trauma that stresses the bone beyond its structural capacity can result in fractures. Bone differs from most other organs in that it retains the potential to regenerate and restore preinjury properties rather than heal by scar tissue of poorer quality than the original tissue. Fracture repair involves a large number of complex coordinated biologic and mechanical interactions that can be conceptualized as involving four major elements, including: (1) mechanical environment, (2) osteoconductive scaffolds, (3) osteogenic cells, and (4) growth factors.2,4 Fracture healing may occur by two basic methods: direct and indirect bone healing. Distraction osteogenesis is a third type of bone healing that is associated with bone lengthening techniques, which are being used more frequently.2,3,5,6 Indirect (secondary) bone healing is the most common type of healing observed in animals and occurs in fractures in which some movement is possible between fracture fragments because of a lack of rigid fixation. Secondary bone healing involves callus formation involving both intramembranous and endochondral ossification. Intramembranous ossification forms bone directly from osteoprogenitor cells and undifferentiated mesenchymal cells in the periosteum away from the fracture site without a cartilage precursor. Endochondral ossification occurs by ossification of cartilage produced by recruitment, proliferation, and differentiation of undifferentiated mesenchymal cells from the periosteum and external soft tissues adjacent to the fracture site.7 The initial stage of healing is characterized by hematoma formation and subsequent inflammation. Although callus formation mimics embryonic bone formation, the presence of inflammation is a major difference, and the interaction between the immune system and the skeletal system is an area of emerging understanding in fracture repair.4,8,8 Motion between fragments produces strain on the tissues attempting to heal the fracture and causes tissue disruption if the strain is excessive. Initially the fracture gap is bridged by tissues that are more stress tolerant, with replacement of each tissue type by a more rigid type of tissue until a rigid bridge is formed between the fragments. Granulation tissue can withstand 100% stretching before failure, whereas fibrous tissue can withstand only 10% and bone only 2% deformation before failure.2 The initial hematoma at a fracture site is replaced by granulation tissue followed by fibrous connective tissue that is replaced by fibrocartilage and then endochondral ossification to produce a bony union. The initial bony bridging callus is woven bone that is remodeled over time to produce compact cortical bone (see Fig. 16-11).2,6 Direct (primary) bone healing is healing that occurs directly between fracture fragments without a cartilaginous stage and no observable callus. Excellent anatomic reduction and alignment of the fracture fragments with rigid fixation are required. The fracture gap must be very small—no more than 150 to 300 µm. The gap is filled initially with fibrous bone followed by remodeling and reconstruction of the haversian systems across the fracture to provide a stronger union. 3,5,5 Distraction osteogenesis results from gradual distraction of the bone segments, often after osteotomy. The gradual widening of the osteotomy gap, ideally 1 mm per day, allows deposition of parallel columns of osteoid leading to formation of lamellar bone within these columns if sufficient stability is present (Fig. 16-1). The goal is to lengthen a bone that is too short as a result of premature growth cessation or to correct a misshapen bone.3,10 Many factors individually or in combination can have a marked effect on the success or failure of fracture healing. The time required to achieve a clinical union adequate to change or remove orthopedic fixation devices varies significantly depending on these factors. Many variables are known to influence facture healing adversely. These include age and weight of the patient, quality of anatomic reduction, stability of fracture, extent of local blood supply, type of fracture, bone involved, presence of infection, iatrogenic interference, systemic diseases such as metabolic and endocrine diseases, pathologic fracture, corticosteroids, and use of nonsteroidal antiinflammatory drugs.2,3,5,11–14 Anatomic reduction with a narrow facture gap will improve the chance of direct or rapid indirect healing. Large fracture gaps or missing bone fragments require more and larger callus formation and a longer time for bridging of the fracture. In addition, anatomic reduction allows the apposition of the bone fragments to enhance the stability of the fracture. Positioning of fracture ends should have at least 50% contact to expect healing of the fracture. However, fracture healing is more likely with anatomic reduction. Anatomic reduction is critical with articular fractures to prevent long-term cartilage damage and degenerative changes from abnormal pressure distribution.2 Stability is a key factor in successful healing of a fracture; motion at the fracture site is the most common cause of poor fracture healing in animals. Motion caused by poor fixation or excessive patient activity is a significant contributing factor. Strain produced by movement in the fracture gap can disrupt the tissues, including new blood vessels required for proper healing. Less rigid fixation results in a larger callus with a prolonged chondral phase and continued new bone formation with later initiation of callus remodeling.15 The effect of mechanical factors on bone healing is well known; however, the detailed mechanisms of mechanotransduction in fracture healing continue to be identified.16 Lack of stability delays healing until more stable tissues can form in the fracture gap and produce sufficient stability for osteogenesis (Fig. 16-2).4,17,17 Viability of the surrounding soft tissues has a significant effect on the ability of bone to heal.3,19,19 Viable adjacent soft tissue provides protection for bone fragments and a source of extraosseous blood supply that is vital in the healing process. Disruption of the normal blood supply to the fracture zone inhibits the repair process. Blood vessels from surrounding tissues are recruited to provide adequate oxygen and nutrients for fracture repair. When the fracture is healed, these extraosseous vessels become dormant as the normal blood supply to the bone is revitalized. Severe damage or loss of surrounding soft tissue decreases the rate of fracture repair and may prevent healing. Fracture fragments that are denuded of soft tissue and bone grafts require adequate stabilization to allow early revascularization of the bone and healing of the fracture. Without revascularization of these bone fragments, healing will not occur. There is increasing emphasis on minimally invasive fracture stabilization techniques that preserve the biologic environment. The technique of minimally invasive plate osteosynthesis shows evidence of more rapid fracture healing compared with more open stabilization techniques in both humans and animals.20–22 The specific bone involved in the fracture can affect the outcome of fracture healing. Some bones have less adjacent soft tissue to supply temporary vasculature for healing. Some small canine breeds have delayed healing of antebrachial fractures with a higher complication rate than larger breeds (Fig. 16-3).23,24 Stabilization of the calcaneus is more difficult because of the normally higher stress on this bone. Infection of bone or surrounding tissue can have a profound effect on healing. Infection can disrupt healing in the fracture gap directly but also indirectly by causing loosening of the fixation device, allowing the fracture to become unstable. The recognition and aggressive treatment of infection is important for successful bone healing (Fig. 16-4). More detailed information on recognition of bone infection radiographically is presented in Chapter 17. Proper selection and application of the fixation device has a significant effect on the rate and success of fracture repair. The fixation device must provide fracture stabilization and not interfere with healing. Common problems include inadequate size and placement of intramedullary pins or insufficient number of plate-associated screws on each side of a fracture that allow fracture instability as well as excessive soft tissue disruption that delays or prevents revascularization (Fig. 16-5). Adequate knowledge, experience, and skills are necessary for the appropriate treatment of bone fractures. 2,3 Miscellaneous factors, including species, breed, age of the patient, nutritional status, and presence or absence of metabolic disease, can affect the duration and success of fracture healing. Some species, such as horses, require longer times for clinical fracture healing because of their greater weight and slower metabolism compared with the average dog. Fractures in younger animals heal faster than those in older animals of the same species.25 Hypothyroidism, hyperparathyroidism, diabetes mellitus, and some paraneoplastic syndromes can delay bone healing.12 An estimated 5% to 10% of the 7.9 million fractures sustained annually by humans in the United States do not result in satisfactory union following initial treatment. The failure of bone healing results in enormous healthcare and socioeconomic costs. This represents a strong economic incentive to develop novel technologies to enhance bone healing.26 The incidence of nonunion fracture in dogs is approximately 3.4%27 with a range of 0% to 6%.28 The incidence of nonunion fracture in cats is 0.85%29 to 4.3%.13 The following brief summary is presented to recognize the importance and diversity of innovative biologic bone healing techniques that are being developed. Many studies published in the last 6 years have added significantly to the knowledge base in the field of biologic bone healing techniques, with potential benefits for enhanced bone repair in humans and animals. Emerging biologic bone healing therapies fall into the following main categories: (1) physical stimulation therapies, including low-intensity pulsed ultrasound and electromagnetic field stimulation; (2) local biologic stimulation therapies, including platelet gel and platelet-derived growth factor, bone marrow cells, prostaglandins, cholesterol statins, bone morphogenetic proteins, and vascular endothelial growth factor; (3) systemic biologic stimulation, including parathyroid hormone, growth hormone, and modifiers of the Wnt intracellular signaling pathway.26,30–34 Low-intensity pulsed ultrasound and extracorporeal shock-wave therapy have been in clinical use since the early 1990s; however, results of controlled and clinical studies have been mixed, and the role of these techniques in the treatment of fractures remains unclear.35–39 Bone grafts have been used for decades, with the use of autologous bone grafts being the gold standard. Autologous bone grafts provide osteoconductive, osteoinductive, and osteogenic characteristics without compatibility problems. However, autologous bone grafts extend surgical time and are of limited supply in smaller animals, and there are potential complications at the harvest site.40,41 Bone allografts are available commercially and may be incorporated into fracture repair to provide osteoconductivity and additional support. Various types of allografts are available and differ in their intended use, with the most common use being associated with repair of large defects and in spinal fusion.40 The development of endoprostheses may replace allograft use because of similar outcomes and easier availability.42 Other osteoconductive biomaterials are available widely and may be grouped into ceramic-based or polymer-based bone graft substitutes. Osteoinductive bone graft substitutes stimulate osteogenic differentiation of local undifferentiated cells and are especially useful for treating nonunion or compromised fractures. Osteoinductive bone graft substitutes include demineralized bone matrix, platelet concentrates, bone morphogenetic proteins, and other growth factors. Osteogenic bone graft substitutes consist of connective tissue progenitor cells capable of differentiating into osteocytes. Bone marrow aspirates are currently the most common source of these cells that can be used alone or in combination with other fracture repair techniques to enhance bone healing.40,41,43–48 Physical examination is an essential first step in the evaluation of fractures and possible complicating factors. Dealing with immediate life-threatening injuries takes precedence over imaging to look for fractures. Careful systematic palpation of the entire skeleton is warranted in patients suspected of having a fracture. Obvious abnormalities must not distract from a thorough examination for less-obvious but significant fractures. Identification of open fractures, spinal fractures, and skull fractures requires careful evaluation with temporary stabilization. Appropriate treatment of open wounds associated with fractures prevents further injury and bacterial contamination that can affect the outcome negatively. Although the overall condition of the patient must be considered, unnecessary delay of treatment is undesirable because delaying fracture stabilization for more than 48 hours after injury is associated with a poorer functional outcome.2,49 Knowledge of normal anatomy and normal variations or aberrant anatomic change is critical for accurate interpretation of images. Normal and accessory ossification centers and normal or aberrant nutrient foramina can mimic fractures.50,51 Anatomic references and skeletal models are invaluable and should be readily available. Radiography remains the most commonly used imaging tool for fracture evaluation in veterinary medicine. Two orthogonal views of the area in question are essential for adequate evaluation of a potential fracture. A single view does not allow complete assessment of the fracture fragments and can be misleading, possibly causing disastrous results. In some patients oblique views are necessary to define or identify a subtle or complex fracture. With minimal displacement, the fracture line must be parallel to the x-ray beam for the radiolucent fracture line to be seen (Fig. 16-6). If the clinical findings indicate a high probability of fracture and no fractures are identified on initial radiographs, oblique radiographs should be made. Small stress fractures or incomplete fractures may not have sufficient displacement immediately following the injury to allow detection. Follow-up radiographs in 7 to 10 days may be needed to detect these fractures as the fracture line becomes more conspicuous. The normal healing process begins with some resorption of the ends of the fracture fragments. Sometimes early callus formation is the only finding that allows identification of a stress fracture. In some patients, other anatomic structures may obscure a fracture on some views, and multiple views may be required to detect the fracture.52 Computed tomography (CT) is especially useful in characterization of fractures in regions with complex anatomy such as the nose, skull, and pelvis (Fig. 16-7). Detail of both cortical and trabecular bone is excellent, and fractures and fissures that are not observed on radiographs are readily apparent in CT images.53,54 In addition, adjacent soft tissue injuries can be observed as well as underlying conditions contributing to a pathologic fracture.55 Postoperative assessment of articular fractures with CT improves the detection of step deformities.56 Scintigraphy is a sensitive method to detect stress fractures and other occult fractures not identified on radiography. Increased uptake of a bone-seeking radiopharmaceutical is related to osteoblastic activity. Bone scintigraphy is very sensitive, being able to detect a stress fracture in an equine metacarpal bone within 24 to 72 hours of injury.57,58 However, its specificity is low because of other diseases that can cause increased radiopharmaceutical uptake. The history, degree of uptake, degree of lameness, and information from other imaging modalities must be considered in determining a probable diagnosis of fracture.57,59,59 Scintigraphy does require patient isolation for radiopharmaceutical clearance after the procedure. Scintigraphy in orthopedic patients is used primarily to identify possible sites of occult fracture or bone lesions not detected by other imaging means. Magnetic resonance imaging (MRI) is the modality of choice for the diagnosis of many musculoskeletal disorders in human beings.1 MRI provides significantly more information than radiography in evaluation of the extent of injury to an open physis and the extent of physeal closure following injury.1 MRI is most notable for its detailed imaging of soft tissues with significantly better soft tissue contrast resolution than CT. MRI is especially useful for detecting abnormal changes in muscles, tendons, ligaments, and cartilage. MRI is sensitive to changes in bone marrow that can aid in the identification of bone lesions that would not be otherwise detected. Radiography is ineffective in the differentiation and identification of soft tissue injuries, and although CT has better soft tissue contrast resolution than radiographs, neither provides the exquisite soft tissue images possible with MRI.55,61 Ultrasound examination of musculoskeletal injuries is becoming more common, primarily for evaluating the soft tissue component. Evaluation of tendons and ligaments with ultrasound is common.62,63 The integrity of bone surfaces can be evaluated with ultrasound with some success in observing occult fractures and sequestra.64 However, sonographic skill and familiarity with the anatomy are required. Although ultrasound is useful in the evaluation of fracture healing, this is not common practice, possibly because of the high-quality equipment and advanced sonographer expertise required.65 Fracture classifications serve to standardize language to improve communication. Some are developed to organize fractures into clinically useful groups that help guide treatment options and prognosis.5,55 Fractures are classified commonly according to location, direction, complete or incomplete status, number of fracture lines, displacement, and open or closed status. Additional descriptive terms are used in combination with the basic classification to further describe a fracture or describe a specific type of fracture.3,52 Location is the first descriptor used in describing a fracture and includes the bone involved and location in the bone. Diaphyseal fractures of long bones may be described with the diaphysis divided into thirds; proximal, distal, or mid-diaphyseal. Metaphyseal fractures of long bones are described as involving the proximal or distal metaphysis. Epiphyseal fractures involve the adjacent joint and physis commonly. If the physis is open, the Salter-Harris classification system is used to describe the fracture (discussed later). Articular fractures consist of any fracture that enters a joint. Important aspects to describe with an articular fracture are extent and location of the articular surface involved and if there are free fragments within the joint. Physeal fractures involving an open physis are described with the Salter-Harris classification system.66
Fracture Healing and Complications
Bone Tissue
Bone Healing
Factors Affecting Bone Healing
Promoting Fracture Healing
Fracture Identification
Fracture Classification
Stay updated, free articles. Join our Telegram channel
Full access? Get Clinical Tree
Fracture Healing and Complications
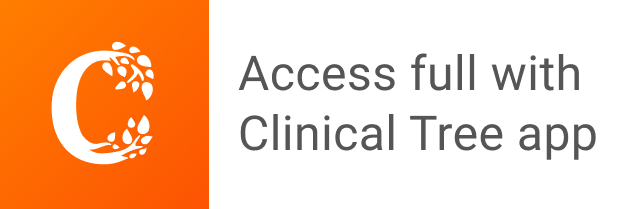