, Monika Hassel2 and Maura Grealy3
(1)
Centre of Organismal Studies, University of Heidelberg, Heidelberg, Germany
(2)
Spezielle Zoologie, Universität Marburg FB Biologie, Marburg, Germany
(3)
Pharmacology and Therapeutics, National University of Ireland Galway, Galway, Ireland
22.1 A View Back to History
The theory of evolution referred to developmental biology ever since it was founded. Classic developmental biology provided many important contributions. Textbooks of classic comparative anatomy, comparative embryology and zoology provided fundamental knowledge about the action and results of evolution. In the last 20 years or so, molecular genetics and studies on expression patterns of genes open new and often unexpected insights into the mechanisms and putative paths of evolutionary changes.
In presenting classic pieces of evidence we confine ourselves to a brief recapitulation of remarks distributed in this book among various sections. Here we will focus on molecular genetic aspects and the molecular “toolkit” the content and meaning of which will be explained with a few selected examples.
22.1.1 “Ontogeny Recapitulates Phylogeny”: Phylotypic Stages, Conserved Paths Versus Novelties
In Chap. 5 (Vertebrates) and Chap. 6 (Human) we pointed out that in all vertebrates in a middle period of their embryonic development a similar basic body construction, a conserved ‘Bauplan’ (construction plan, body plan), can be recognized. Conserved characteristics are: dorsal neural tube, notochord, somites, branchial pharynx with gill pouches, cartilaginous pharyngeal arches (gill arches), from 4 to 6 gill arteries, and a ventral heart. In their subsequent ontogeny these basal characteristics develop quite differently in class-, family- and eventually in species-specific ways, reflecting the phylogeny.
Vertebrates of all taxonomic groups transitorily pass developmental stages reminiscent of their common ancestor. Temporarily it appears as if the tiny human embryo (1–20 mm in size) would be about to develop into a fish, subsequently into a salamander, a reptile, a monkey and finally into a human being (see Figs. 6.24 and 6.9). Such observations prompted anatomists and zoologists of the eighteenth and nineteenth century such as Carl Ernst von Baer and Ernst Haeckel to emphasize these astonishing similarities and to formulate ideas and hypotheses which culminated in Haeckels much debated “basic biogenetic law” – which in fact is a rule – stating ontogeny be an abbreviated form of phylogeny (see Sect. 6.6). However different the very early and the very late developmental stages may be, in the middle period a phylotypic stage (Figs. 5.9 and 6.8) can be recognized in which the embryos are amazingly similar to each other (Fig. 6.17). The concept of phylotypic stages has first been applied to vertebrates but phylotypic stages can also be defined in other groups. In insects, for example, the stage of the extended germ band is considered to represent the phylotypic stage. In molluscs it is the veliger larva, in echinoderms the gastrula or the pluteus larva can be considered to be phylotypic though, or because, these pre-metamorphic stages display bilateral symmetry thus representing the ancestral primordial trait of the phylum.
Back to vertebrates: Already the fathers of developmental biology not only emphasized common traits but also pointed out that it is just the earliest phase of development in which development proceeds highly differently and in class-specific patterns, being in mammals very different compared to amphibians. For instance in human embryogenesis, very early preparations are made for developing the placenta, even before the embryo proper is formed. In the blastocyst the outer layer becomes an extraembryonic precursor of the placenta, called the trophoblast (Greek: trophein = to nourish) as it serves to take up nutrients provided by the uterus of the mother. Accordingly, the embryo does not arise from the entire ball-shaped embryo, called the blastula in amphibians and blastocyst in mammals; instead in the mammalian blastocyst the embryo actually forms from a small area in the interior (see Fig. 6.5). Such differences reveal adaptations to habitats as different as the pond and the womb.
22.1.2 Functional and Gene-Regulatory Constraints Restrict Possibilities of Large Evolutionary Changes
In all vertebrates a transitory notochord is formed prior to the vertebral column. This apparent detour has a practical function. The notochord assists in mechanically stretching the head-tail axis and it is an indispensable emitter of development-organizing signals. It orchestrates the formation and subdivision of the spinal cord (Fig. 16.1), summons the cells of the somites to migrate and to assemble around the notochord where they form the vertebral bodies (Fig. 10.6). The notochord even assists in determining the position of inner organs such as liver and pancreas. Ontogenetic inputs and presetting cannot arbitrarily be modified; the processes are too complex and must take place in a sequence of progressive steps.
Another example of constraints: In all vertebrates capable of flight, pterosaurians, birds, and bats, wings could only be developed at the expense of the forelimbs. The gift of grace of another extra pair of extremities remained reserved for mythological figures such as dragons and winged unicorns, and the celestial angels. The range of attainable morphologies is confined.
Fossil records provide evidence that the basic traits of all recent body plans already existed in the Cambrian period about 540–480 million years ago. They thus arose from a putative common ancestor that already existed previously. Fossils in the form of imprints resembling recent medusae are known from the Precambrian (Vendium, 640 million years ago) but fossil records do not provide information on how these constructions once arose, simply because residues of soft tissue do not persist or merely persist as imprints in rock. Clues to the earliest history of life can only be deduced from the developmental pathways and the inventory of conserved genes of recent animals.
22.2 New Possibilities of Knowledge Acquisition: Evo-Devo Biology und Its Basis
22.2.1 “Evo-Devo” Biology Based on Molecular Genetics Aids in Elucidating Evolution by Comparing Gene Sequences, Expression Patterns and Regulatory Networks
The remarkable congruency of basic developmental mechanisms and gene expression patterns in organisms belonging to different taxa gave a new lease of life to an area of research that became known as “evo-devo” biology and increasingly attracts attention. Though awareness that ontogeny partly recapitulates phylogeny enabled and stimulated synergies between developmental and evolutionary biology for a long time, only the comparative analysis of gene expression patterns and regulatory networks merged these complementary disciplines on a broad level.
With entire genomes sequenced from a yearly increasing number of animals that belong to different taxa it is becoming apparent that type and complexity of a body plan does not correlate with the total number of genes. Evolution generates duplications of key developmental genes, change of function of the duplicated copies, and altered integration into regulatory networks. For example changes in the body plan may be caused be the acquisition of new binding sites for transcription factors (as in case of lens proteins, see below) or by an altered time span or time sequence by which signalling molecules, receptors or other proteins are produced and active.
Comparative analyses including many different species show that a molecular “toolkit” has existed since the early phase of history. Already at the level of the Cnidaria this toolkit contains almost the entire stock of instruments that enabled the triploblastic animals to develop an amazing diversity of body plans. (But of course, as this basal toolkit as such did not per se give rise to triploblasty in cnidarians, the kit must contain additional tools in the triploblastic clades.) According to the hypothesis of a common toolkit some genes are expected to fulfil similar tasks in morphologically different organisms. There is hope that by detecting such conserved genes and identifying their function it might be possible to define an ancestral “zootype” – an ancestral prototype animal expected to be similar to the common ancestor of all multicellular animals, the Metazoa.
In contrast to classic developmental biology with only few but intensely investigated model organisms evo-devo biology analyses a broad spectrum of recent taxa and species that are positioned close to branching points in the phylogenetic tree or whose position is a matter of debate. Alternatively, or in addition, the function of developmentally relevant proteins is compared across the entire animal kingdom. By comparing the expression patterns of key marker genes and the regulatory interactions of gene products, it is possible to identify traits of common ancestors at different evolutionary levels. In parallel, in comparing gene sequences by means of computer programs (algorithms), phylogenetic relationships can be deduced, compared and matched with existing pedigrees construed on the basis of morphological or biochemical traits (Fig. 22.1).
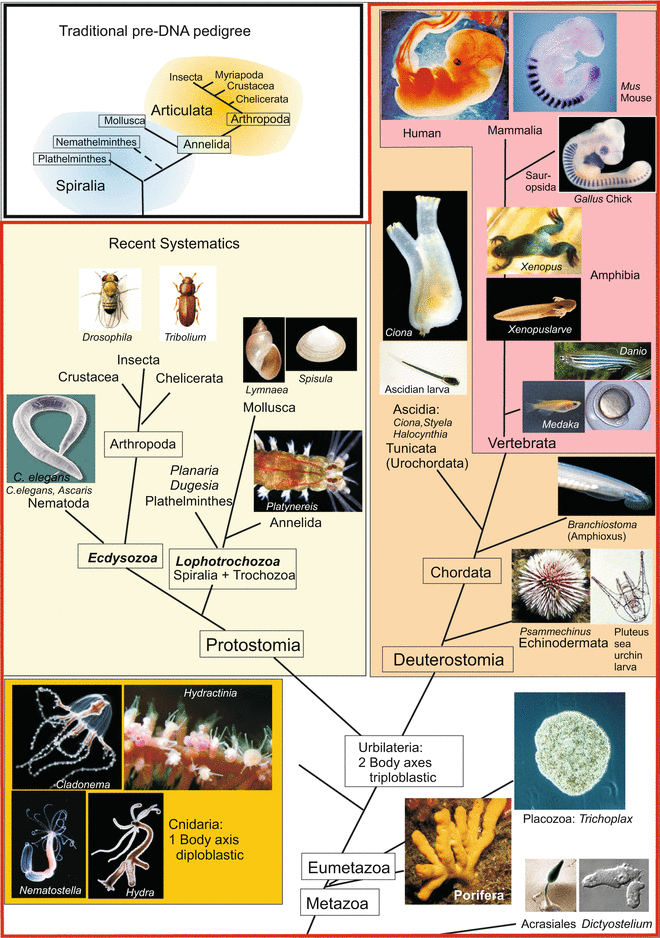
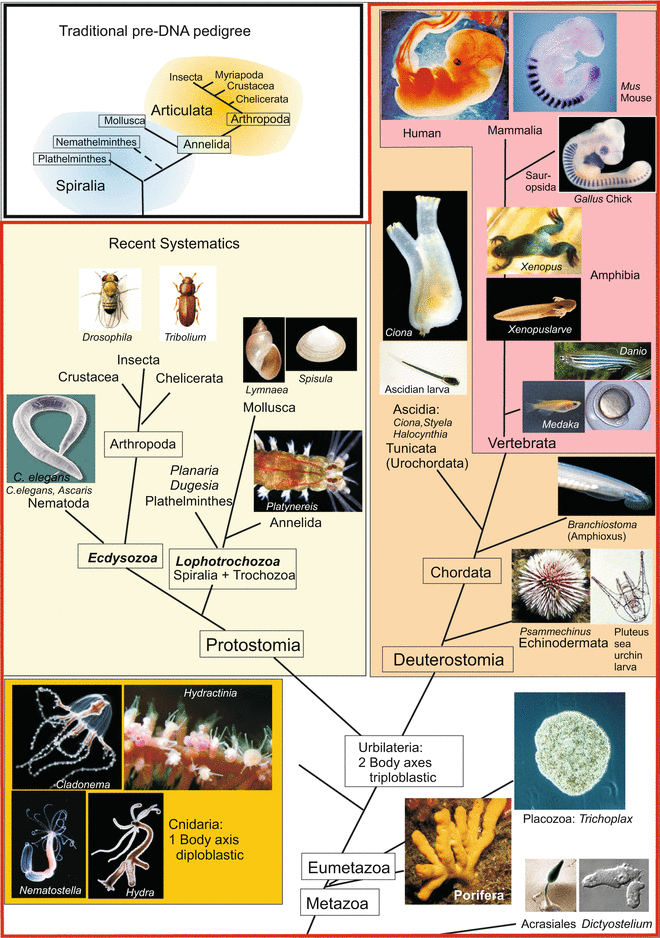
Fig. 22.1
Phylogenetic tree of the animal kingdom with the positions of model organisms mentioned in this book. The insert at the upper left shows a traditional phylogeny with Articulata as superorder comprising segmented animals including the segmented spiralian Annelida as bridge unit
Functionally highly conserved gene products are, as a rule, integrated in conserved molecular mechanisms of regulation and control similar structures. On the other hand, molecular biology also reveals convergences by showing that the morphology and function of a structure can be attained and ensured by different ways. Thus, in the course of evolution, different molecules may have been utilized to generate, for example, a refractive eye lens.
Before we consider the procedure and results of evo-devo research by discussing actual examples we first summarize the basic assumptions underlying the theory of evolution and discuss problems the researcher is confronted with, whether he/she is trained in molecular biology or/and in zoology.
22.2.2 Homologies Based on Morphology and Homologies Based on Molecular Genetics Are Ascertained Using Different Criteria
Evolutionary changes have their origin in changes in the genome. Relationships and changes in morphology and biochemistry should be reflected in the genome and in the spectrum of the encoded proteins. These, in turn, are the building blocks which bring about the changing morphological traits in ontogeny and the eventual lasting phenotype. Thus, the discovery of the Hox genes and their putative proto-Hox precursors in all multicellular animals (Metazoa) investigated so far pointed to a parallel molecular and morphological developmental sequence correlated with the increasing complexity of body plans (see Sect. 22.4).
Homology in Morphology. When morphologists speak of homology they mean affiliation of a structure to an evolutionary developmental sequence. Homologous anatomical structures in various organisms derive from a common original stem structure. Criteria for homology in the sense of traditional morphology/anatomy are:
1.
The criterion of location of a structure within the fabric of neighbouring structures
2.
The criterion of the special quality (e.g. functioning as a sensory organ or a gonad)
3.
The criterion of continuity when a structure underwent a change. Structures altered in evolution should be connected with the ancestral structure by intermediates.
4.
Criterion of cell type. Not explicitly listed in traditional treatises is a criterion that currently links molecular biology with morphology most closely: the criterion of cell type. Intuitively and self-evidently nobody would homologize a muscle with a bone or a brain. And one may subsume the criterion of cell type under the criterion of specific quality. But, for example, what is the evolutionary origin of various sensory cells? The hypothesis has been proposed that photoreceptors once derived from odorant receptors. In both cell types molecular receptors consisting of seven-pass transmembrane proteins of the same protein family are essential (Chap. 11). Retinal of photoreceptors can be considered to be a permanently attached ligand of an odorant receptor, a ligand that is converted into the active state by light.
Homology in Molecular Biology When molecular biologists and biochemists speak of homology they mean high sequence identity in genes or polypeptides, respectively. Nobody doubts that genes displaying sequence identity over many hundreds or thousands of nucleotides derive from a common primordial gene. In genes or proteins, respectively, with low sequence similarity but similar function, the three-dimensional folding structure of the protein might have been preserved; but in this case convergence must be also taken into consideration. Moreover, recombination of DNA modules by exon shuffling may cause partial homology to several different genes or proteins (see below Sect. 22.3.1) and thus make reconstruction of a phylogenetic tree a difficult task.
22.2.3 Homology of Organs Is Not Always Based on Homologous Genes and Homologous Genes Can Contribute to Building Non-Homologous Organs
Does homology at the level of genes not per se imply homology at the level of the phenotype? Unfortunately it does not! Four examples will provide proof.
Example 1
Non-homologous genes for homologous structural elements – cartilaginous versus ossified skeletal elements. When, in the course of evolution, a skeletal element is optimized or experiences a successive change in shape and function, its stock of molecular components can change, just as it does in ontogeny. A cartilage that undergoes ossification exchanges its entire chemical composition, even related molecules such as the collagen isoforms are replaced (cartilage has collagen type II whereas bone has type I).
No morphologist or embryologist would designate the bony femur and its cartilaginous ontogenetic precursor as convergent structures because in the course of life the composition of the building material changes and this restructuring uses other genes than in the original embryonic edition. Cartilage and bone, whether primarily formed with embryonic sets of genes or secondarily reformed with adult sets of genes derive in evolution from the same ancestral origin. Homologous structures can in the course of ontogeny and phylogeny exchange their molecular components and this can reflect an evolutionary sequence, such as the transition from Chondrichthyes (cartilaginous fishes) to the Osteichthyes (bony fishes).
Example 2
Non-homologous lens proteins for eye lenses. The lenses of the vertebrate eyes are homologous in the sense of morphology, meaning they are deemed to be derived from a common ancestral primordium. The biochemist on the other hand, who analyses the components of the lenses in different species, discovers a surprising number of different proteins which confer glassy transparency to the lens. What has been said about the exchange of bone for cartilage applies also here: a function can be assumed by other materials and thereby become optimized for a special purpose.
Basically, many low molecular weight proteins are suited for constructing a lens because most proteins, at least in their native state, do not absorb light in the visible range of wavelengths and thus are transparent. A stable protein conformation is essential. Denaturation and precipitation of α-crystallin is the main cause of cataract, i.e. clouding of the lens, in humans. Furthermore, the protein concentration along the optical axis must vary to confer adequate refractory properties to the lens.
Lens proteins in different animals can belong to different protein families, and not only in animals which are commonly placed at distant positions on the phylogenetic tree but also in vertebrates. In the vertebrate eye changing combinations of up to 11 different proteins are found that collectively are called crystallins. Yet, these crystallins are not members of a single protein family but are quite different in their amino acid sequence and thus encoded by non-homologous genes. Most crystallins are sequence related to certain enzymes or stress proteins (chaperones). Some crystallins are active enzymes, while others lack activity but show homology to known enzymes. Those from birds and reptiles are related to lactate dehydrogenase (in crocodiles called δ-crystallin) and argininosuccinate lyase, those of mammals to alcohol dehydrogenase and quinone reductase.
In the convergently evolved lenses of invertebrates other sets of proteins are used. Cephalopods (squids) for example use glutathione S-transferase and aldehyde dehydrogenase documenting that in fact many different proteins are suited for this purpose. In retrospect not surprisingly, the corresponding genes are expressed in other tissues as well. Evolution recombines proteins in new patterns in response to functional needs. Constraints demand only that a transparent and refractory structure must arise.
For the construction of structures classified as homologous in terms of morphology different macromolecules can turn out to be suited, and newly recruited macromolecules can replace previously used macromolecules.
Example 3
Homologous genes for non-homologous structures. Macromolecules counted by molecular biologists as homologous are often expressed in different tissues and organs which in the understanding of morphologists are not homologous. The HOX-D13 gene for example is in the mouse embryo expressed exclusively in somite No. 7, but also at the tip of the outgrowing tail and at the tip of the outgrowing limbs. Homology at the level of genes and proteins is not in each and every case congruent with homology at the level of tissues and organs!
Example 4
Homologous genes in structures with cryptic homology. The comparison of expression patterns occasionally aids in recognizing a common origin of organs of which the homology was controversial or not expected. A good example is the common genetic origin of the central nervous system in insects and vertebrates. Here it is the large number of homologous genes expressed in similar patterns that provide evidence for cryptic homology (Chap. 16, Fig. 16.13). Another example are photoreceptors and eye types the development of which is triggered by key genes conserved across the entire animal kingdom, namely Pax6 and/or sine oculis, and the subsequent differentiation of these photoreceptors is controlled by several common downstream genes (see Fig. 12.19, and below Sect. 22.7). If development of complex organs in various animals belonging to different taxa is triggered and controlled by the same set of conserved homologous genes, sole convergence can be excluded. Convergence is based on a series of random genomic changes which cannot by chance be identical at all relevant sites in the genome.
Controversial and unexpected homology of structures can be verified by a compendium of cooperative homologous genes.
To conceive evolutionary coherence and relationship it is not possible to rely on one single trait or one single gene. In the worst case one could focus on a gene that late in evolution was imported by a retrovirus across species boundaries and now pretends genealogical relationships. In the euphoria of the early molecular genetics founding period some conclusions were drawn prematurely. Molecular genetics had to learn the same lesson as had the morphologists of the old school. The reconstruction of a phylogenetic tree on the basis of one single trait or one single gene can easily be misleading.
22.2.4 Orthologous and Paralogous Genes and Structures Must Be Evaluated Separately
In general terms, homology means: two entities have a common origin in the history of life. For example in various vertebrates, say zebrafish, grass frog, and hummingbird, the spinal column and the forelimbs (pectoral fin, foreleg, and wing) are homologous to each other. In a restricted sense one speaks of orthologous structures, meaning structures derived from a common ancestor and found in different species. Yet, within one and the same individual the somites and vertebral bodies are also homologous repetitions of the same basic theme. Likewise, in tetrapods forelimbs and hindlimbs are variations of a basic structure – one speaks of serial homology or of paralogous structures.
In parallel the molecular geneticist finds homologous genes in different species and taxa. The Hox gene labial, for instance, exists in Drosophila and the mouse, and likewise the gene Antennapedia. Both genes are members of the Hox cluster and arose from a common primordial gene, that was serially duplicated, and in annelids, arthropods and vertebrates is found in the anterior part of the Hox cluster (Figs. 22.2 and 22.3). One speaks of
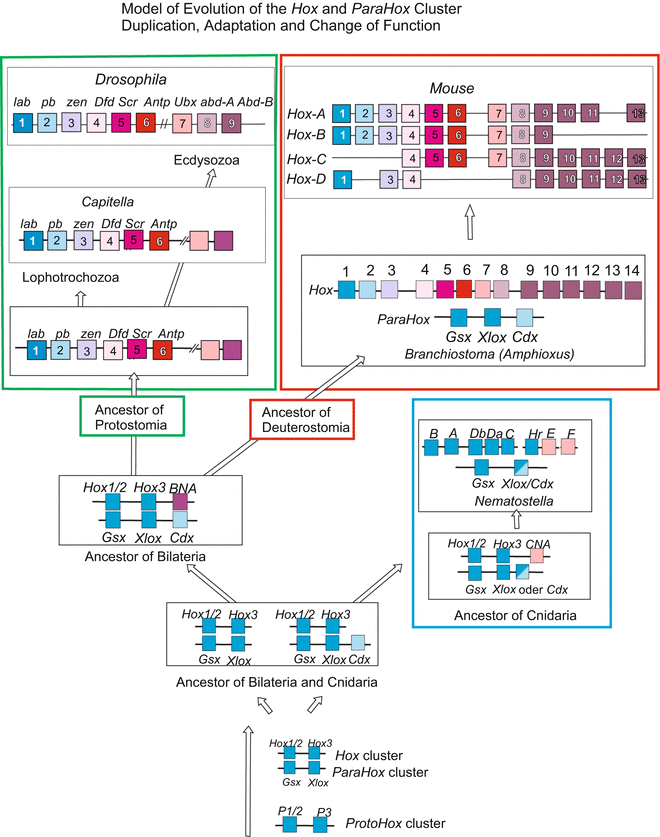
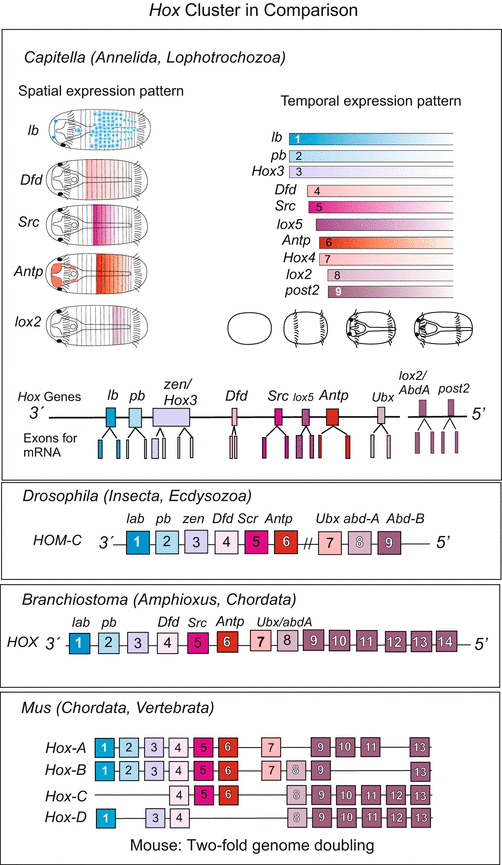
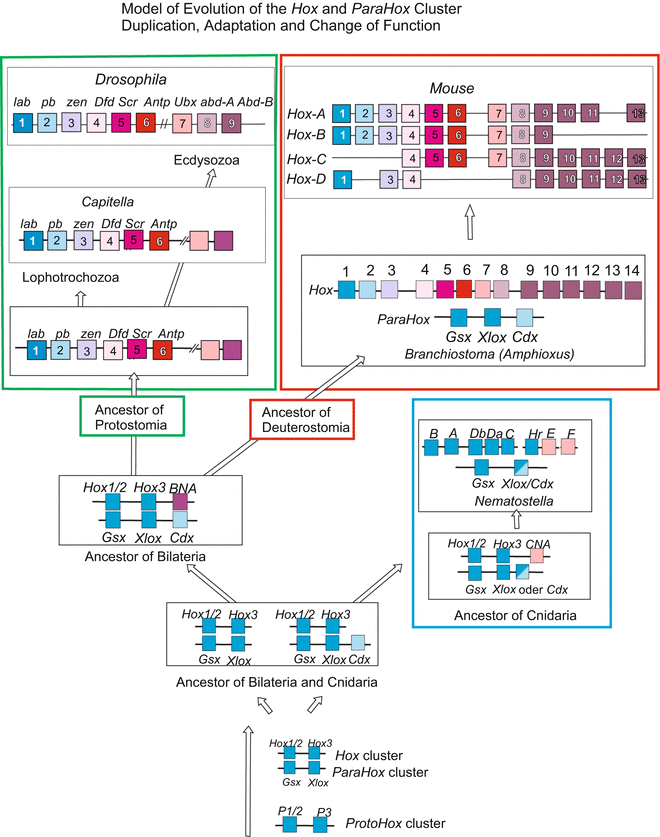
Fig. 22.2
Hypothesis concerning the evolution of Hox genes from simple proto–Hox precursors. The proto–Hox genes persist in extant animals. After Chourrout et al. (2006), compiled by WM
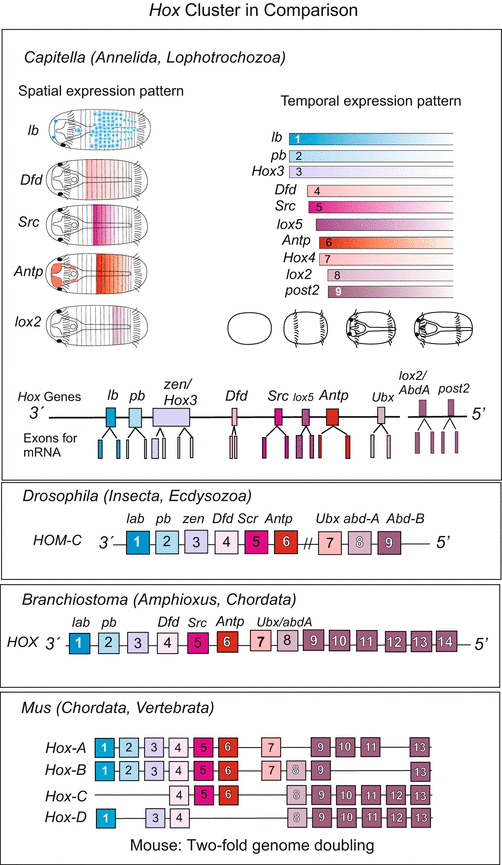
Fig. 22.3
Hox cluster in various organisms. Note: The almost complete congruence in number and sequence of the Hox genes in the annelid Capitella and Drosophila documents that already the common ancestor possessed a complement of 9–10 Hox genes. Despite difference in detail, their expression pattern in developmental stages of the annelid Capitella corresponds to insects. The general principle of colinearity is retained (after Fröbius et al. 2008a). In mammals the Hox cluster exists fourfold, one of several indicators that in the evolution of mammals the complete genome was duplicated twice. Compiled by WM
orthologues, when the genes in question derive from a (putative) ancestor common to two or more divergent lineages of descendants and, therefore, are found in the genomes of different species. One speaks of
paralogues, when the genes were multiplied within the genome of an ancestor and are found in one and the same individual at different locations of its genome. The duplicates may become different with time and undergo cooption for different tasks, or turn into non-functional pseudogenes by loss-of-function mutations.
Genetic relationships between organisms can reliably only be reconstructed when orthologous genes are compared! To reconstruct a phylogenetic tree of the Hox cluster, for example, the labial gene of a given species must be compared with the labial gene of other species, or the Antennapedia genes with Antennapedia genes of other species, but not labial genes with Antennapedia genes. (A comparison of paralogues can be used to reconstruct the branching routes within the Hox cluster). Orthologous key genes with conserved functions, in particular orthologous master- or selector genes, point to common principles in the control mechanisms.
22.3 Novelties Derived from Legacies in the Genetic Program
22.3.1 Novel Genes Arise from Gene and Genome Duplication and Mutation-Induced Alteration of the Duplicates
If natural relationships and evolutionary changes are reflected in the genomes we may expect that genes exist which vary to some extent from species to species but are conserved on the whole. On the other hand, we also expect novelties, for humans are neither hummingbirds nor insects.
Gene synthesis de novo is not known up to date (at the utmost genes might arise from non-coding sequences, see framed text in Sect. 22.3.3). So how can novelties emerge?
Basic mechanisms creating something new may be comprehensible when we consider how conserved gene clusters with several paralogs and how multidomain proteins arose. Based on the crystalline examples above, we may also envision how non-homologous proteins can be used to optimise the function of homologous structures.
Gene duplication. The duplication of a primordial gene by imprecise crossing-over (Fig. 22.4 or by means of transposons (see textbooks of genetics) generates subsequent to duplication new perspectives for natural selection. One copy is left to perform the original function under the pressure of natural selection. The second copy can be varied by mutations and assume modified or even new functions. Examples are the different rhodopsin alleles (Fig. 22.5) that are expressed in the cones of our retina and enable colour discrimination.
Fig. 22.4
Gene duplication by inprecise crossing over
Fig. 22.5
The different opsins arose by duplication of one ancestral opsin gene; the variants allow analysis of wave length composition (colour) of light. Composed by WM
Multiple duplications: In case of the Wnt genes and other multigene families, and in case of the Hox genes such duplications with subsequent mutations in the duplicates happened several times leading to paralogous genes. In the Hox gene cluster all the descendants of the primordial gene still code for transcription factors but mutation-mediated changes in the DNA-binding domain enabled activation of different sets of downstream genes by different members of the Hox family. Presumably, the entire Hox cluster arose from two proto–Hox genes (Fig. 22.2). As said above, the members of this group are classified as paralogues if compared with other members along the cluster; they are classified as orthologues if one distinct member of this group is compared with the corresponding member in another species. (However, the introduced classification and nomenclature of the Hox genes was based on the comparison of the DNA-binding homeodomain, and does not exclude more extensive differences in other regions of the genes).
Genome duplication. Duplication of genetic information can happen not only at the level of single genes but also at the level of chromosomal segments, chromosomes and the whole genome. In the mouse and in humans the Hox cluster is present four times, each cluster located on a different chromosome (see Figs. 12.16 and 22.3). Because Branchiostoma (Amphioxus), a representative of the Cephalochordata and as such closer to the origin of the phylum Chordata, possesses only one single cluster. The fourfold quantity in mammals indicates that during the evolution of vertebrates the entire genome was duplicated twice. Duplication of the entire genome is, for example, possible if in the first meiotic division of primordial germ cells the chromosomes are not distributed to two daughter cells, and diploid gametes result. In the very rare cases this happens in both sexes, a tetraploid organism such as Xenopus laevis can arise. Moreover, many polyploid organisms are known, or polyploid tissues in diploid organism such as liver cells in humans.
Point mutations. It is not necessarily a huge event that will have a profound effect. Already the emergence of a new allelic variant can have a strong impact on developmental processes. Imagine an enzyme that degrades a morphogen. A new variant of the enzyme, working quicker or slower, can make a morphogen gradient steeper and shorter, or flatter and longer. This can lead to a shortening or an extension of body regions (compare Fig. 4.34). The implication of new gene variants, however, cannot be deduced from the primary sequence but must be elucidated through functional and biochemical investigations.
22.3.2 Novelties Arise from Recombination of Existing DNA Sequences, for Instance by Exon–Shuffling
Sequence analysis reveals in many proteins a modular composition with similarity to different functional domains of other proteins. In particular, a modular composition is evident in multimodal growth factor receptors, which combine ligand-binding and enzyme activity. A growth factor receptor of the FGFR family (see Fig. 11.8) for example possesses an extracellular ligand-binding domain, a transmembrane domain, various intracellular docking sites, and a kinase domain. The partial sequence similarity to receptors of other types and families suggests that these proteins are composed of modules. In the course of replication and recombination new genes were created in evolution by recombining segments of already existing genes. A plausible and widely accepted hypothesis proposes exon–shuffling as a mechanism of recombination. As a rule, genes of eukaryotes are interrupted by several interspersed introns. Exon-intron boundaries frequently correlate with those of protein domains. Through recombination of initially separate exons highly complex multimodal protein might have arisen (a model of proteins consisting of segments derived from recombined DNA segments are the antibodies, see Fig. 18.8). According to recent reports about the origin of the growth factor binding receptor tyrosine kinases (RTK) first events of recombination took place already in the common ancestor of multicellular animals and their sister group, the choanoflagellates. Such processes warn us about using partial sequences in genes and proteins as sole indicators of homology. On the other hand comparing partial sequences found in extant organisms enable reconstruction of the evolution of modular proteins using algorithms of bioinformatics, and to correlate the result with phylogenetic trees constructed based on morphological, biochemical and genetic criteria.
22.3.3 Horizontal Gene Transfer: Many DNA Sequences Are Imported from Foreign Genomes
In the genome of all species investigated so far DNA sequences of apparently foreign origin are found. Frequently foreign (heterologous) DNA is transferred by viruses from one organism into another organism, also irrespective of borders between species or even phyla. Such sequences persist in the form of transposons or sequences partially reminiscent of transposons. Their viral origin is evident or suggested by characteristic subsequences. Even in non-functional remnant sequences partial sequences coding for viral envelope proteins (gag, env) or other typical viral sequences (pol, ORF) or characteristic palindromic flanking sequences remain traceable testifying their viral origin. According to quantitative estimations transposons and related sequences sum up to about 45 % of the human genome. They are commonly looked upon as junk DNA, but among the sequences imported into our genome sadly there also are cancer-generating oncogenes (Chap. 19). Beneficial for humans is, on the other hand, the likely cooption of transposons in lymphocytes. The many short sequences framed by palindromic sequences are used to program the variable, antigen-binding parts of B-cell receptors and hence of antibodies by somatic recombination (Fig. 18.8). The same applies for the variable domains of the T-cell receptors. Apparently, transposons were put into service of the adaptive immune systems during evolution of the vertebrates. In biotechnology, transposons are regularly used as vectors to produce transgenic animals.
Surprises are to be expected in evolutionary biology. In 2009 the emergence of a new gene was reported in mouse, which had been generated from a previously functionless intron in a long process that included random mutations. Researchers concerned with the less-noticed phylum of Cnidaria report numerous sequences of prokaryotic origin included in the cnidarian genome. Which mechanisms caused or allowed their uptake and integration into the host genome is unknown.
22.3.4 The Species-Specific Stock of Genes Is Also Determined by Loss of Genes
When the first genomes were sequenced, an astonishing number of conserved genes was found to be shared by the fly Drosophila, the round worm Caenorhabditis elegans and man. On the other hand in the human genome genes were detected that also were found in other mammals but not in the fly or the worm. These genes therefore were counted as mammalian-specific. Yet, many of the allegedly mammalian or vertebrate-specific genes were subsequently identified in the genomes of various members of the phylum Cnidaria (Acropora, Nematostella, Hydra, Hydractinia) as well as in the genome of the annelid Platynereis dumerilii. Apparently these genes were already present in the common ancestor of the Cnidaria and Bilateria but were lost in the branch leading to the Ecdysozoa including Drosophila and C. elegans.
Once more it must be emphasized that phylogenetic relationships can be reconstructed reliably only by comparing multiple genes from many different taxa. These restrictions must be taken into account in any evo-devo projects and reconstructions of phylogenetic trees.
22.4 The Common Toolkit
In this section we will show, using four selected examples, that the origin of conserved development-regulating genes dates back prior to the Cambrian period 550 million years ago when the basic body plans of all recent animals already were established. By taking the example of a few gene families it becomes evident that small changes in the genetic regulation or integration in genetic networks can have strong effects on the manifestation of molecular and morphological traits.
If one compares the genomes of all metazoa available to date, from sponges and cnidarians up to the mammals, one finds that genes coding for transcription factors are highly conserved among the genes relevant for developmental control. For example the eye development-triggering transcription factors PAX6 and SINE OCULIS can be traced back to the origin of the Metazoa. This will be explained in Sect. 22.7.
22.4.1 The Wnt/β-Catenin System Acts as a Conductor in Early Axis Specification Across the Metazoa
In many animal groups the WNT signalling system is concerned with the establishment of the primary body axis, or in bilaterally symmetric animals, the establishment of one of the three body axes, the longitudinal anterior-posterior axis. Signalling centre is the posterior pole (Fig. 22.6). Moreover, the system is concerned with the control of stem cells in all animals investigated.
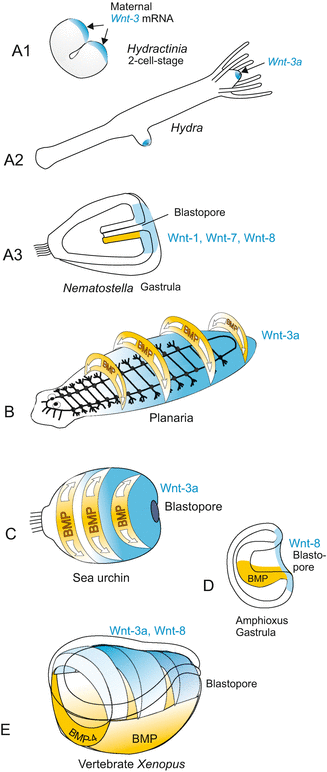
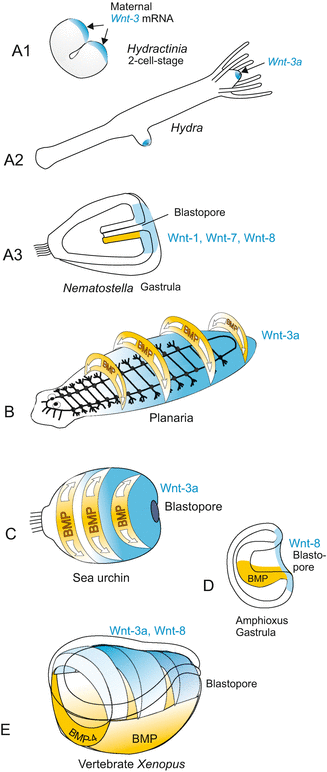
Fig. 22.6
WNT expression in the establishment of one/the body axis in various organisms. Composed by WM
If one wants to understand how body plans developed from the early to the complex animals it is reasonable to look at animal taxa that early in evolution diverged from the main lineage of the tree leading to bilateral symmetric animals. It is obvious that presence of a conserved gene in representatives of early side branches and also in the Bilateria lineage indicates its presence in the inventory of the last common ancestor that existed before the branches diverged. Among the taxa that branched off early in evolution are the Porifera (sponges), the Placozoa represented by the tiny two-layered Trichoplax, and the Cnidaria with Clytia, Hydra, Hydractinia, the coral Acropora, and the sea anemone Nematostella. The majority of multicellular animals share an indirect development with a simple motile ciliated larva. This larva has an anterior end pointing in the direction of forward movement, and has an elongated body. Whether this longitudinal axis is called antero-posterior axis, oral-aboral axis, or head-tail axis is a matter of convention and tradition. It is this main longitudinal body axis that is established under the regime of the canonical WNT/β-catenin system (Fig. 9.5).
In this context the question is of interest whether the oral-aboral body axis of the Cnidaria corresponds to the antero-posterior axis of higher evolved bilaterian animals, and therewith, whether the head of Hydra, sea anemones and of corals is equivalent to the head of annelids, insects, or vertebrates. This question is discussed in Box 22.2.
The Wnt/β-catenin signalling pathway (see Fig. 11.6), once detected as a signalling system that is required for correct outgrowth of wings in Drosophila, turned out to be essential for the establishment of an embryonic body axis in all animals investigated with respect to the genetic basis of axis formation, except in Drosophila itself. WNT signalling molecules are expressed early in embryogenesis – in part by maternal, in part by zygotic genes. In previous Chapters we referred to the examples of the hydroid Hydractinia (Fig. 9.5), sea urchin (Fig. 4.6) and Xenopus (Figs. 5.18, 5.19, and 10.4) and found a common principle: The WNT system directs and patterns the body axis that extends from the blastopore to the opposite pole (Fig. 22.7). The head-tail axis (a-p axis) in vertebrates can only be implemented correctly when WNT is emitted from the posterior pole at the blastopore, and on the other hand at the anterior pole anti-WNT factors neutralize the WNT signals thus enabling head formation. Working in the opposite direction, WNT and anti-WNT gradients share responsibility in the subdivision of the body into head, trunk, and tail. However, when we compare representatives of different taxa we see an important difference. While in triploblastic animals WNT is expressed with maximum intensity at the posterior pole and excluded in the head region, in Cnidaria we see the obviously inverse situation: Wnt is expressed at the head pole. Such an inversion is not detected when we take the blastopore as point of reference and invert the polyp upside down: wnt expression indicates that Hydra is standing on its head.
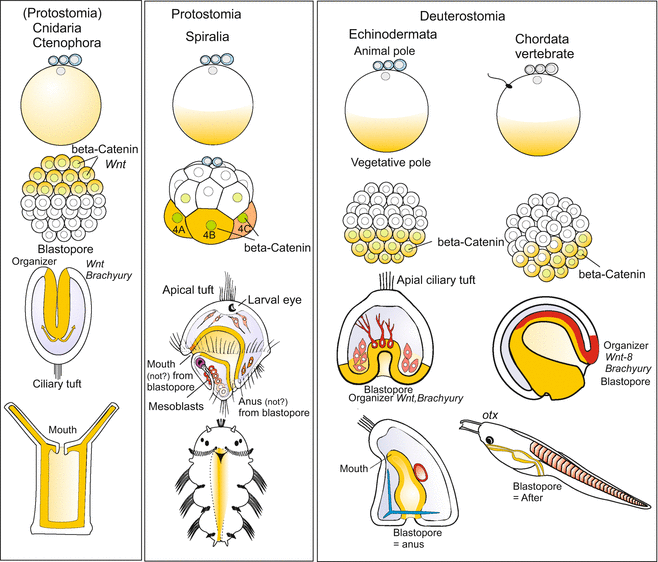
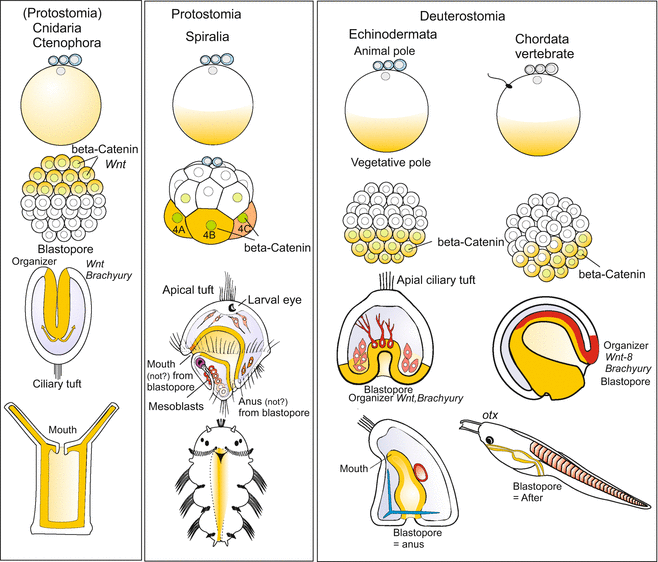
Fig. 22.7
Position of the blastopore (gastrulation) and development of the longitudinal body axis in various representatives of the animal kingdom. Note that in Cnidaria the place of gastrulation is at the pole where the polar bodies form and which is designated animal pole in other animals. Here also the head (hypostome) will arise. By contrast in the bilateral organisms gastrulation takes place at the vegetal pole or close to it. After Martindale and Hejnol (2009), and other authors, compiled, modified and extended by WM
Unexpectedly, already early in phylogeny an ample collection of WNT signalling molecules occurred through repeated duplication of the wnt gene. Already in Cnidaria 11 wnts are present! The successive and overlapping expression of all these WNT ligands along the body axis in Nematostella points to a potential function in the subdivision of the body column into functionally different zones (Fig. 22.8). The canonical WNT system apparently is a system that was acquired early in the history of life for specification and regionalization of the primary body axis. In triploblastic animals this system was supplemented or replaced (Drosophila) by HOX transcription factors.
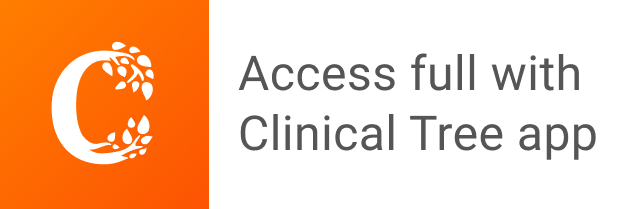