Patrick Boerlin The definitions of virulence and pathogenicity, and the difference between a pathogenic and a non‐pathogenic bacterium have been the subject of many controversial discussions. The increasing importance of opportunistic infections in debilitated and immunodeficient patients, which blur the distinction between pathogens and commensals, is contributing to make the situation even more complex. The most widely accepted definitions of pathogenicity and virulence are “the capacity of a microbe to cause damage in a host” and “the relative capacity of a microbe to cause damage in a host,” respectively (Casadevall and Pirofski 1999). However, the capacity of a microorganism to cause damage is not a function of just the pathogen. It also depends on the host and on the circumstances of the encounter between the host and the pathogen (Méthot and Alizon 2014). To complicate things further, the ability of a pathogen to cause damage resulting in disease in a host is usually the consequence of interactions among several bacterial factors. Defining which factors are really responsible for causing damage to the host and are therefore virulence factors has also remained a controversial issue (Casadevall and Pirofski 1999), resulting in the delineation of a variety of more or less distinct virulence factor categories (Wassenaar and Gaastra 2001). The origins and circumstances leading to the emergence of most new pathogens are unclear and hypothetical. Among the many publications that describe and review the emergence of new pathogens, most are dedicated either to human pathogens or to rapidly evolving RNA viruses. Only a few bacterial pathogens of animals have emerged in the recent past, and the related literature is very limited. However, researchers have developed general theoretical frameworks on the emergence of human pathogens that are of relevance and can be adapted for bacterial pathogens of animals (Woolhouse and Gaunt 2007). Pathogenicity and virulence may be the result of a variety of encounters and adaptations between a host and a microorganism. Different levels of interaction in the evolution of a microorganism toward a full‐blown infectious pathogen can be distinguished and represented in the form of a pyramid with highly transmissible pathogens at the top (Woolhouse and Gaunt 2007). This evolutionary pyramid can integrate at every transition stage the ecological “source–sink” model, in which a source reservoir of organisms keeps refilling a new, less‐adequate, ecological compartment – the sink (Sokurenko et al. 2006). The first stage (Figure 2.1) in the emergence of a pathogen (base of the pyramid) is the encounter with a new host or new body compartment of an already existing host. If the bacterium possesses or acquires a sufficiently broad repertoire of survival mechanisms, an infection with this “fortuitous” pathogen may take place (stage 2). Such a newly emerged “pathogen” may be using tools that evolved to promote its survival in a completely different environment/host and circumstances, resulting de facto in virulence. Legionella pneumophila is an example of such a “fortuitous” pathogen, which uses complex transmission strategies and a variety of virulence factors that evolved for survival in protozoans (Boamah et al. 2017) but which also cause damage in humans. Figure 2.1 Theoretical frame for the emergence of new pathogens and evolution of virulence. Bacterial pathogens may stop their evolution toward increased virulence (orange arrows) at any stage or revert toward lower virulence (blue arrows). Bacteria from the environment or from other animal species most frequently enter the pyramid at its bottom, but entry at any stage is possible, although less likely. Similarly, a pathogen may completely lose its pathogenicity at any stage. The color intensity is indicative of the likelihood of changes in virulence. The triangular shape of the arrows is representative of the “source‐sink” nature of transition between stages. The interaction of a “fortuitous” pathogen with a new host or body compartment is in most cases a dead end for the microorganism, but repeated contact if frequent or intensive enough may allow a host to encounter a rare mutant with improved tools for survival and multiplication in this new environment. If it results in survival and persistence of the microorganism in some hosts, followed by return to its original environment or transmission to another susceptible host, then a new infectious pathogen is born with the capacity of being transmitted from one individual to another (stage 3). Depending on transmissibility, persistence, and exposure, this can lead to disease outbreaks of variable amplitude. Finally, if transmission becomes frequent enough, the pathogen may cause epidemics and spread broadly across host populations (stage 4). It is thought that many emerging pathogens have followed this path of evolution. However, the pyramid can be entered at any level and there is no necessity for a microorganism to follow this path to the top. Indeed, the majority of bacterial pathogens do not end up causing large epidemics. This model of emergence of new pathogens should not be understood as a one‐way evolution as the movement of a bacterium in the pyramid may go down as well as up. Both evolutionary changes leading to the acquisition of new characteristics in a pathogen (survival, transmissibility, invasion, and ability to cause damage) and changes in its environment or in its potential host population may trigger a move between virulence levels. The former is the main subject of this chapter. The latter includes increased contact between potential pathogens and new hosts through ecological changes, changes in vector populations (climate change, hygiene), and changes in host populations (e.g. emergence of immunodeficient populations, increased stress, or host density, changes in behavior promoting transmission, and genetic changes affecting susceptibility). Tick‐borne diseases and Lyme disease, in particular, are examples of emergence of old pathogens caused by ecological changes and expansion of their vector populations (Sonenshine 2018). Although it does not take much to imagine many similar situations in animal populations and the potential for the emergence of new pathogens in both companion and farm animals, the “context‐dependent” nature of virulence evolution (Diard and Hardt 2017) makes its general modeling and prediction very difficult. Pathogenicity is just one among numerous strategies available to microorganisms to remain competitive in the fight for limited resources in a frequently hostile environment. The evolution of pathogens does not always lead to a continuous increase in or maintenance of virulence. The deleterious effects of exploitation of the host may ultimately also affect the microorganism itself. Thus, pathogens have to evolve ways to take advantage of their host without compromising their own survival and multiplication (i.e. to maintain a high fitness level in terms of population biology). Common wisdom would suggest that the general evolutionary trend of pathogenic microorganisms would be toward commensalism, and that high virulence levels could be a sign of recent colonization of a new host by a microbial species. The longer a microorganism persists and associates with a host species, the less virulent it would become, until it reaches the climax of commensalism. Unfortunately, this rather simple reasoning does not fit with the natural history of many pathogens. A positive relationship between virulence levels and transmissibility of microorganisms has been postulated to accommodate the inconsistencies of the common‐wisdom theory. Experimental and observational studies show that highly virulent microorganisms are often readily transmissible, whereas less virulent ones are less easily or less frequently transmitted tend to support this hypothesis, known as Ewald’s hypothesis or trade‐off hypothesis. However, the reality of infectious disease is still more complex, and this simple theory is not able to explain every case of virulence and transmission patterns observed in the field (Alizon and Michalakis 2015). Many researchers have questioned its validity, but it is likely that including more case‐specific variables into each model of virulence evolution would reconcile the trade‐off hypothesis with the biological realities seen in the field (Alizon et al. 2009; Cressler et al. 2016). Host variability and host population structure are important factors influencing the evolution of virulence (Alizon et al. 2009; Ekroth et al. 2021). This variability is very broad and extends from the within individual host variability (the potential anatomic locations of a pathogen within a host) to the between‐host variability, which may encompass the variability between host species for multihost pathogens (Pfennig 2001; Woolhouse et al. 2001). The mechanisms governing the evolution of a microorganism toward a specialized or a generalized multihost pathogen are complex. The adaptation to a new host may sometime encompass a trade‐off between the development of virulence in a new host and the loss of virulence and fitness in a previous host, and multiple models have been developed to explain the adaptation and transition between hosts (Sheppard et al. 2018). Alternatively, pathogens may develop such a constant strong genetic polymorphism that individuals adapted to new hosts and new situations will be present in each population, thus broadening the host range of the pathogen (Pfennig 2001; Woolhouse et al. 2001). A pathogen may also evolve many different virulence mechanisms and express them only under certain conditions, depending on host and environment (a phenomenon called polyphenism). Thus, not only the virulence factors of a pathogen per se but also their regulation is subject to evolutionary constraints (Pfennig 2001; Coombes 2013). Competition between microorganisms for a host or an ecological niche within the host is another important factor driving the evolution of virulence of some pathogens. Finally, the influence of coevolution of a host and pathogen remains poorly studied. Nevertheless, examples of field studies on coevolution are emerging, both at the population level (Maillard et al. 2008) and at the molecular level (Stavrinides et al. 2008; Shames et al. 2009). In light of the preceding, it is clear that the mechanisms governing the evolution of virulence are extremely complex and varied. Much more research is needed before we can seriously envisage the development of strategies to manipulate the evolution of virulence. Three major mechanisms are available to bacteria to maintain variability and adaptability to new environments (Figure 2.2): local sequence change through mutations, DNA rearrangement, and DNA acquisition (lateral or horizontal gene transfer, HGT). Although this is not an absolute rule, mutations and internal rearrangements are thought to result mainly in stepwise adaptations and to be mostly involved in microevolution and in fine tuning (genetic drift). On the contrary, HGT has frequently been associated with more drastic changes and in major macroevolutionary steps in the lifestyle and virulence of pathogens (genetic shifts). Mutation rates are not always constant in the life of a bacterial population. In the presence of environmental changes or other stresses, a diversity of mechanisms may increase the mutation rates, such as induction of the SOS system of bacteria or emergence of mutator strains defective in the control of DNA replication fidelity (Sundin and Weigand 2007). Although this phenomenon has been suggested to play a role in the adaptation of pathogens to the stressful host environment and in the evolution of virulence, some methodological biases may have led to overestimation of its role (Frenoy and Bonhoeffer 2018). A few publications supporting the potential role of elevated mutation rates in the evolution of bacterial virulence can be found for instance for pulmonary infections with Pseudomonas aeruginosa (Hogardt et al. 2007), urinary tract infections with Escherichia coli (Labat et al. 2005), and host adaptation of Salmonella (Nilsson et al. 2004). These elevated mutation rates seem to be particularly important in the case of long‐term chronic infections. However, their significance for the clinical outcome for patients has also been questioned (Oliver 2015). Figure 2.2 Main molecular pathways of virulence evolution. The modes of new DNA acquisition and associated genetic elements are shown on the left. The modalities of pathoadaptation are shown in the center and right. Acquisition of new characteristics is denoted in red and losses in blue. ICEs, integrative and conjunctive elements; PAIs, pathogenicity islands. Rearrangements, including the formation of deletions nicknamed “black holes” in the genome of pathogens, represent another mechanism in the adaptation of pathogens to new hosts. The loss of so‐called antivirulence genes, which leads to “black holes,” is not stochastic as genome decay (see section on genome reduction) and does not lead to similar extensive genome size reductions. The loss of functions encoded by these antivirulence genes may, under particular circumstances, be of advantage to the pathogen. The transition of Yersinia pseudotuberculosis to Yersinia pestis and the emergence of Shigella spp. and enteroinvasive E. coli from non‐pathogenic E. coli are examples in which antivirulence genes were actively counterselected. In both instances, the loss of some metabolic functions was associated with an increase in virulence. As genome sequences accumulate, more and more of these antivirulence genes will be discovered (Bilven and Maurelli 2012). Bacterial species vary greatly in their ability to accept foreign DNA and to integrate it into their genome. A broad diversity of genetic behaviors can be observed in natural populations of bacterial pathogens, ranging from completely clonal (species in which HGT is not able to disrupt significantly the overall tree‐like evolutionary structure resulting from the binary cell division) to panmictic populations (populations with frequent exchange and rearrangement of genetic determinants resulting in an overall network‐like evolutionary structure) reminiscent of eukaryote populations with a sexual reproduction cycle (Feil and Spratt 2001). Despite this apparent range of evolutionary behaviors, advances in population genetics using molecular biology tools such as comparative genomics, show that bacteria rely mainly on HGT for the major steps in their evolution (Pallen and Wren 2007), in particular, with regard to the evolution of virulence (Ziebuhr et al. 1999). HGT is mediated by three major transfer mechanisms (Gillings 2017): transformation (direct uptake of naked DNA by competent bacteria), transduction (DNA transfer and recombination through bacteriophages), and conjugation (transfer of plasmid and/or chromosomal DNA through mating between a donor and a recipient bacterium). All three transfer mechanisms are known to occur for virulence determinants (Figure 2.2). Nanotubes and membrane vesicles are two other relatively newly discovered mechanisms of HGT. The extent of their role in the evolution of pathogens remains unknown (Gillings 2017). Although HGT represents a very important first step in the evolution and adaptation of a pathogen to their host, it is not the end of the road. After transfer, the foreign DNA has to be stabilized, replicate efficiently, and be expressed adequately. Finally, the newly acquired characteristics have to be fixed in the new bacterial host population. The genetic background of the new host may play an important role in these later steps (Escobar‐Paramo et al. 2004). In the case of transformation, the first step of stabilization is predicted to occur through homologous recombination rather than through illegitimate incorporation, thus usually limiting the range of new genetic information introduced into the recipient to genes from closely related bacteria. Through their internally encoded replication and integration functions, phages, plasmids, conjugative transposons, and integrative and conjugative elements (Burrus and Waldor 2004) seem to be able to transfer and stabilize new genes from more distant donors and to play a more important role in the evolution of virulence. Because of the selective advantage of coordinated regulation, genes transferred in clusters or as part of whole operons have more chance than single genes to be fixed in a new host (Lawrence 1999). Mutations, rearrangements, and recombinations are subsequently responsible for fine tuning the expression of the acquired virulence characteristics (Ziebuhr et al. 1999). HGT and stabilization of transferred genetic material favor the emergence of clusters of genes. Hot spots within genomes, where the newly acquired genes tend to accumulate more frequently, contribute to avoid disruption of the structure and functionality of the core genome function in a species (Touchon et al. 2009). One of the major concepts that emerged during the past two decades with regard to clustering of horizontally transferred genes is that of genomic islands (Hacker and Carniel 2001). The term “island” was first used for clusters of virulence genes from uropathogenic E. coli called pathogenicity islands (PAIs). They were later shown to be a widespread phenomenon in bacterial pathogens in general (Gal‐Mor and Finlay 2006). PAIs, which represent a specific subgroup of genomic islands (Juhas et al. 2009), encode a variety of virulence factors such as toxins, superantigens, adhesins, invasins, iron uptake systems, type III (T3SS) and type IV (T4SS) secretion systems, and many other effectors that modulate the behavior of host cells. They are found in a large variety of pathogens (Hacker and Kaper 2000). Except for their presence in pathogens and their specific virulence genes, PAIs share most general characteristics with other genomic islands (Hacker and Kaper 2000), which are listed in Table 2.1. Based on these characteristics, a large number of bioinformatics tools have been developed to identify genomic islands through in silico analysis of bacterial genomic sequences (reviewed in Bertelli et al. 2019). The mechanisms of horizontal transfer of PAIs are still not well understood and may be multiple. The presence of bacteriophage integrase genes or their remnants in PAIs and their propensity to be inserted in the close proximity of tRNA genes suggest that PAI transfer may be related to phage transduction. However, examples relating HGT of genomic islands to bacteriophages, plasmids, conjugative transposons, and integrative and conjugative elements have been described, and comparative studies of integrase genes suggest that many genomic islands may represent independent mobile genetic elements unrelated to phages and other classical mobile elements (Boyd et al. 2009). Analysis of the evolutionary path of some PAIs and their flanking regions suggests that homologous recombination may also play an important role in the dissemination of genomic islands within related host organisms, in addition to site‐specific recombination (Schubert et al. 2009). Table 2.1 Common characteristics of pathogenicity islands.
2
Evolution of Bacterial Pathogens
What are Pathogens and How Do They Emerge?
Bacterial Fitness and Virulence
Sources of Genetic Diversity, Population Structure, and Genome Plasticity
Pathogenicity Islands
Characteristic
Origin or putative function
G + C content and sometime codon usage different from the core genomic DNA
Marker of foreign origin through horizontal gene transfer
Mosaic structure
Remnant of past recombination events and sign of successive adaptation to host(s)
Association with tRNA genes
Hot spots for integration and coregulation
Frequent location on chromosome
Stabilization
Relatively large size (10–200 kb)
Coordination of expression
Encoding one or several virulence factors
Adaptation to host(s)
Usually only in pathogenic species or clones
Adaptation to host(s)
Direct repeats at both ends
Remnant of integration events, maintenance of plasticity and mobility
Cryptic/decaying or functional mobility factor genes
Remnant of integration events, maintenance of plasticity and mobility
Frequent presence of phage‐related genes
Remnant of integration events, maintenance of mobility
Often unstable or mobilizable
Adaptability to new environments and hosts
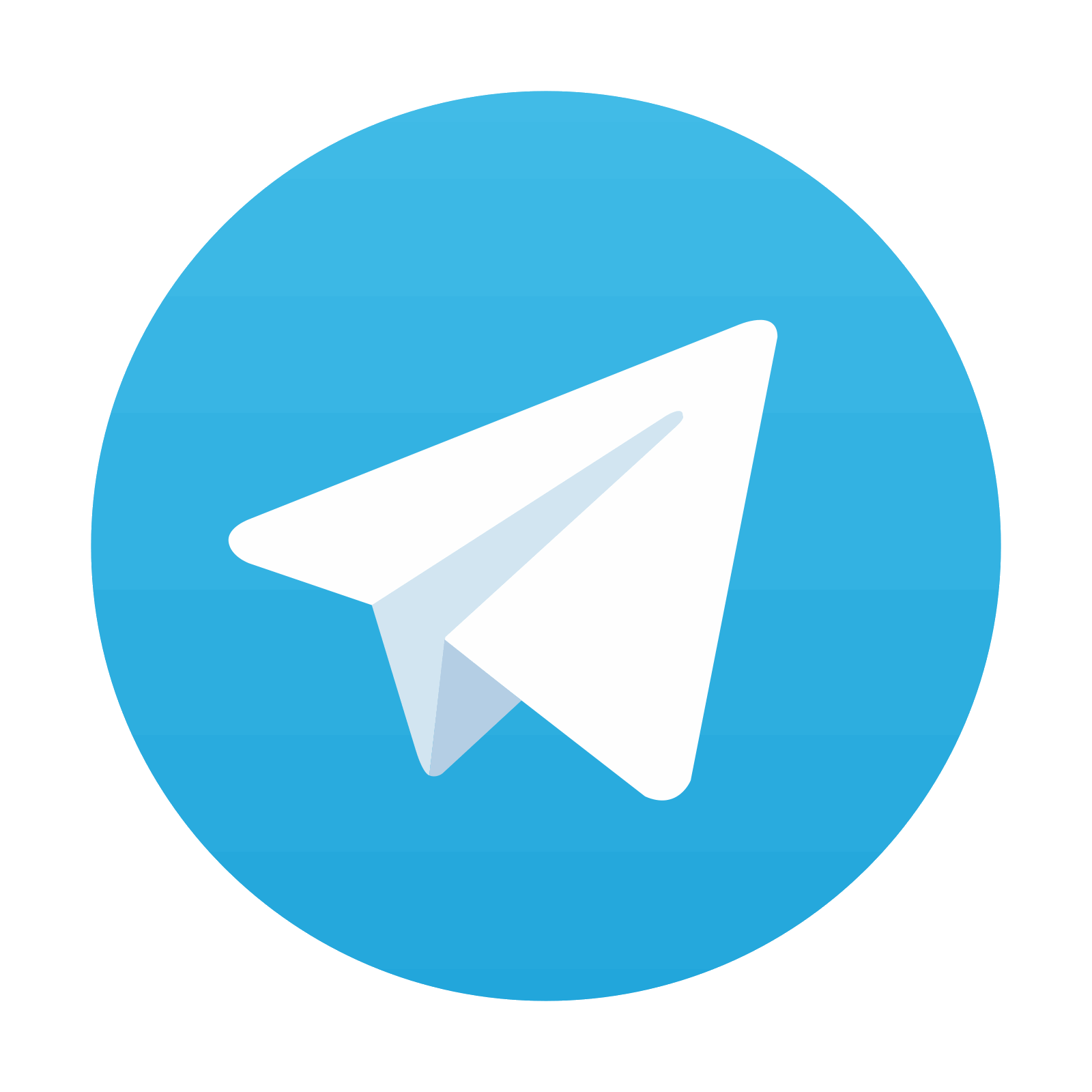
Stay updated, free articles. Join our Telegram channel
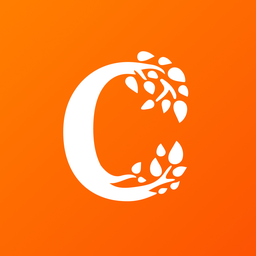
Full access? Get Clinical Tree
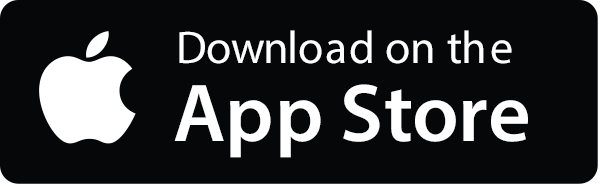
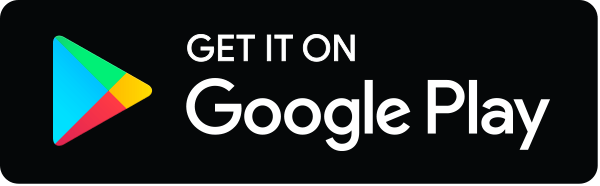