Chapter 4 Evaluation of Erythrocytes
Normal Erythrocytes
Erythrocyte Morphology
Erythrocytes from all mammals are anucleated, and most are in the shape of biconcave discs called discocytes (Figs. 4-1, 4-2).205 The biconcave shape results in the central pallor of erythrocytes observed in stained blood films. Among common domestic animals, biconcavity and central pallor are most pronounced in dogs (Figs. 4-3, 4-4), which also have the largest erythrocytes. Other species do not consistently exhibit central pallor in erythrocytes on stained blood films. The apparent benefit of the biconcave shape is that it gives erythrocytes high surface area : volume ratios and allows for deformations that must take place as they circulate. Erythrocytes from goats generally have a flat surface with little surface depression; a variety of irregularly shaped erythrocytes (poikilocytes) may be present in clinically normal goats (Fig. 4-5). Erythrocytes from animals in the Camelidae family (camels, llamas, vicuñas, and alpacas) are anucleated, thin, elliptical cells termed elliptocytes or ovalocytes (Fig. 4-6). They are not biconcave in shape and are minimally deformable.437 Erythrocytes from birds (Fig. 4-7), reptiles, and amphibians are also elliptical in shape, but they contain nuclei and are larger than mammalian erythrocytes. Blood cells in salamanders are the largest recognized (Fig. 4-8).
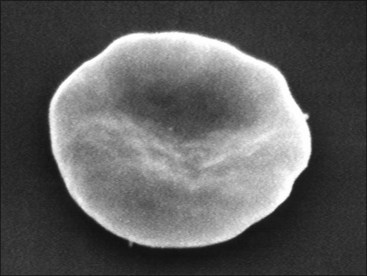
FIGURE 4-1 Scanning electron photomicrograph of a normal horse erythrocyte called a discocyte.
From Stockham SL, Harvey JW, Kinden DA. Equine glucose-6-phosphate dehydrogenase deficiency. Vet Pathol. 94;31:518-527.
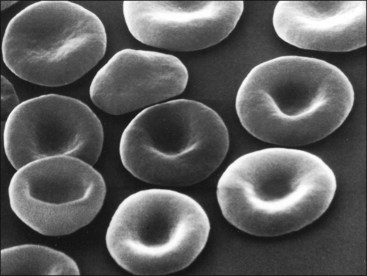
FIGURE 4-3 Scanning electron photomicrograph of dog erythrocytes (discocytes).
Courtesy of K. S. Keeton and N. C. Jain.
Erythrocyte Functions
Each hemoglobin tetramer is capable of binding four molecules of O2 when fully oxygenated. The initial binding of a molecule of O2 to a monomer of tetrameric deoxygenated hemoglobin facilitates further binding of O2 to the hemoglobin molecule. The changing O2 affinity of hemoglobin with oxygenation results in a sigmoid O2 dissociation curve (Fig. 4-9), when the percent saturation of hemoglobin with O2 is graphed against the Po2. The steepness of the middle portion of the curve is of great physiologic significance because it covers the range of O2 tensions present in tissues. Consequently a relatively small decrease in O2 tension in tissues results in substantial O2 release from hemoglobin. The overall affinity of hemoglobin for O2 is decreased by increasing H+, CO2, temperature, and, in most mammals, 2,3-diphosphoglycerate (2,3DPG). There is a direct correlation between body weight and the O2 affinity of hemoglobin in whole blood (lower body weight, lower O2 affinity) when various species of mammals are compared.205
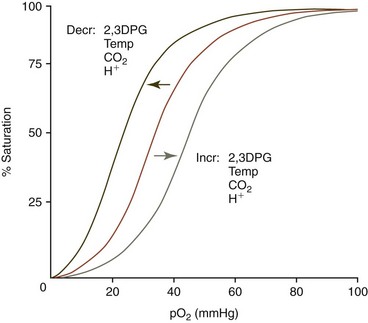
FIGURE 4-9 Hemoglobin-oxygen dissociation curve and factors influencing the position of the curve.
From Harvey JW. The erythrocyte: physiology, metabolism, and biochemical disorders. In: Kaneko JJ, Harvey JW, Bruss ML, eds. Clinical Biochemistry of Domestic Animals, 6th ed. San Diego, CA: Academic Press; 2008:173-240.
The O2 affinity of fetal blood is greater than that of maternal blood except in the cat. Differences in fetal versus maternal O2 affinity may potentiate O2 transport from the mother to the fetus. However, the fetus is subjected to low arterial O2 tensions, and the increased O2 affinity of fetal blood is likely needed to more fully saturate hemoglobin with O2.205
The ability of plasma to carry CO2 is small, but the carbonic anhydrase reaction in erythrocytes increases the CO2-carrying capacity of blood 17-fold by rapidly converting CO2 to carbonic acid (H2CO3). The H2CO3 spontaneously ionizes to H+ and bicarbonate (HCO3−). The HCO3− diffuses out of the cell down a concentration gradient and chloride (Cl−) moves in (chloride shift) to maintain electrical neutrality. These processes are reversed at the lungs. Some CO2 is also transported bound to hemoglobin as carbamino groups. Deoxyhemoglobin binds about twice the CO2 that oxyhemoglobin does.205
Hemoglobin is the major protein buffer in blood. Deoxyhemoglobin is a weaker acid than oxyhemoglobin. Consequently, when oxyhemoglobin releases its O2 in the tissues, the formation of deoxyhemoglobin results in increased binding of H+. Hemoglobin buffers the effects of H2CO3 and allows for the isohydric transport of CO2. Hemoglobin also buffers organic acids produced during metabolism.205
Erythrocyte Biochemistry
Membrane Structure and Function
The erythrocyte membrane contains a phospholipid bilayer with molecules of unesterified cholesterol intercalated between fatty acid chains. Phospholipids can move in various ways and contribute to membrane fluidity. Glycolipids are located on the outer layer of the membrane, with carbohydrate groups extending outward. Some blood group antigens are glycolipids, with their specificity residing in the carbohydrate moieties (see Chapter 6 for a discussion of clinically significant blood groups).205
Membrane proteins consist of integral membrane proteins that penetrate the lipid portion, often spanning the bilayer, and skeletal proteins that form or attach to the internal surface of the lipid bilayer. Glycoproteins associated with the membrane are integral membrane proteins with the carbohydrate residues extending from the outside surface of the cell membrane. They carry erythrocyte antigens and function as receptors or transport proteins (e.g., band 3 is an anion transporter). The membrane skeleton is composed of various proteins located in a lattice-like arrangement on the inner surface of the erythrocyte membrane. This meshwork is attached to the membrane by binding to transmembrane proteins. The membrane skeleton is a major determining factor of membrane shape, deformability, and durability. It is in a condensed configuration in intact cells and can be stretched considerably without rupturing.205
Inherited membrane defects can result in abnormally shaped erythrocytes with shortened erythrocyte life spans and variable degrees of anemia. Band 3 deficiency in cattle results in marked spherocytosis with membrane instability and severe anemia.232 Hereditary elliptocytosis has been reported in one dog with protein 4.1 deficiency and another with mutant β-spectrin.122,205 Neither dog with elliptocytosis was anemic, but the protein 4.1-deficient dog had a reticulocytosis, indicating a shortened erythrocyte life span. Hereditary stomatocytosis occurs in multiple dog breeds, but the specific membrane defects have not been reported.205
ATP Generation
Mammalian erythrocytes lack nuclei; therefore they cannot synthesize DNA or RNA. They also lack ribosomes, mitochondria, and endoplasmic reticula; consequently they have no Krebs cycle or electron transport system and are unable to synthesize proteins or lipids de novo. Glucose is the primary substrate for the energy needs of erythrocytes from all species except the pig, where inosine appears to be the major substrate. Mature erythrocytes depend on anaerobic glycolysis for energy (Fig. 4-10). The ATP generated by glycolysis is needed for the maintenance of erythrocyte ionic composition, shape, and deformability and for limited synthetic activities such as glutathione synthesis. Hypophosphatemia results in decreased erythrocyte glycolytic rates and decreased ATP generation. Hemolytic anemia resulting from hypophosphatemia has been reported in diabetic cats and in a diabetic dog following insulin therapy, in a cat with hepatic lipidosis, and in postparturient cattle and buffaloes.109,205 Deficiencies of rate-controlling enzymes in glycolysis also result in insufficient ATP generation and shortened erythrocyte survival. Pyruvate kinase (PK)-deficient dogs and cats have mild to severe regenerative hemolytic anemia. Phosphofructokinase (PFK)-deficient dogs have compensated hemolytic anemia plus sporadic episodes of intravascular hemolysis and hemoglobinuria.203
2,3DPG Pathway
2,3DPG binds to hemoglobin and reduces the affinity of hemoglobin for oxygen in erythrocytes from most mammals. It is produced from a side pathway of the anaerobic glycolysis pathway. No net ATP is generated when molecules traverse this DPG pathway. The formation of 2,3DPG in erythrocytes is stimulated by increased blood inorganic phosphate (Pi) concentration and increased blood pH, both of which stimulate anaerobic glycolysis. 2,3DPG is the most abundant organic phosphate in the erythrocytes of most species but its concentration is low in erythrocytes of Felidae (including domestic cats), Bovidae (cattle, sheep, and goats), and Cervidae (deer).415 Animals with high erythrocyte 2,3DPG concentrations, including dogs and horses, have the potential to alter their hemoglobin O2 affinity to meet their metabolic needs. The significance (and, in some cases, the appropriateness) of alterations in 2,3DPG in disease states is not always clear. Erythrocyte 2,3DPG concentration increases in some anemic animals, and the resultant decrease in hemoglobin O2 affinity would seem to be beneficial.205 Increased 2,3DPG has also been reported in erythrocytes from horses with hypoxic conditions.173 In the case of severe hypoxic hypoxemia, the response might be detrimental because hemoglobin cannot be fully saturated.241 High-altitude camelids (including llamas, alpacas, guanacos, and vicuñas) have erythrocytes with high hemoglobin oxygen affinity, even though their erythrocytes have relatively high 2,3DPG concentrations, because their hemoglobin exhibits low reactivity toward 2,3DPG.377 The P50 for greyhound erythrocytes in whole blood is lower than that for mongrel dogs, yet the groups have similar 2,3DPG concentrations.458 The cause of this difference remains to be determined, but it could reflect a low reactivity to 2,3DPG. It is suggested that the higher hematocrit found in greyhound dogs may represent a compensatory response to a higher oxygen affinity of hemoglobin in this species.
PFK deficiency inhibits glycolysis above the DPG pathway, resulting in markedly decreased 2,3DPG concentrations, which makes dog erythrocytes alkaline-fragile. Episodes of intravascular hemolysis occur when PFK-deficient dogs develop alkalemia secondary to hyperventilation.203
Oxidant Injury
Animals are exposed to low levels of oxidants in their environments and from normal metabolic processes in the body. Reactive oxygen species and reactive nitrogen species are formed as products of normal cellular metabolism. When they are generated at higher concentrations in disease states, these free radicals (and the even more potent oxidative metabolites that they produce) can overwhelm protective systems within the body, producing cellular injury and/or destruction.492
Oxidative reactions can damage hemoglobin, enzymes (sulfhydryl groups especially), and the membrane lipids of erythrocytes. Methemoglobin forms when hemoglobin iron is oxidized from the +2 to the +3 state. Heinz bodies are inclusions that form within erythrocytes following the oxidative denaturation of the globin portion of hemoglobin. Membrane damage can result in intravascular hemolysis or erythrophagocytosis and shortened erythrocyte life spans.205
Protection against Oxidant Injury
NADPH generated in the pentose phosphate pathway (PPP) provides electrons for protection against oxidants. It is needed to maintain glutathione and thioredoxin in their reduced states, and it is important in maintaining catalase in a functional form. Defects in the PPP can render erythrocytes susceptible to endogenous and exogenous oxidant injury. Glucose-6-phosphate dehydrogenase (G6PD) is the rate-controlling enzyme in the PPP. A persistent hemolytic anemia with eccentrocytosis has been described in an American saddlebred colt with less than 1% of normal G6PD activity.452
Reduced glutathione (GSH) is a tripeptide containing a highly reactive sulfhydryl group that may act nonenzymatically as a free radical acceptor to counteract oxidant damage. GSH also functions as an electron donor in various reductive enzyme reactions including glutathione peroxidase (GPx), phospholipid hydroperoxide glutathione peroxidase, glutathione S-transferase, and glutaredoxin. Following oxidation, glutathione forms a disulfide (GSSG) that can be reduced back to GSH by the flavin adenine dinucleotide (FAD)-dependent glutathione reductase (GR) enzyme, using NADPH as the source of electrons (see Fig. 4-10). Horses with erythrocyte FAD deficiency have markedly reduced GR activity, decreased GSH concentration, and prominent eccentrocytosis.203,212
Selenium acts as an antioxidant when incorporated as selenocysteine at the active site of a wide range of selenoproteins, including GPx, phospholipid hydroperoxide glutathione peroxidase, and thioredoxin reductase in erythrocytes.73 Heinz body hemolytic anemia has been reported in selenium-deficient cattle grazing on St. Augustine grass.327 Catalase is an enzyme that can catalyze the conversion of H2O2 to water and O2 without using energy.
Recent studies suggest that peroxiredoxins may be more important in protecting against H2O2 than GPx or catalase.285 Oxidized peroxiredoxins are regenerated using reduced thioredoxin, and oxidized thioredoxin is reduced by NADPH using thioredoxin reductase.277 Ascorbate functions as an antioxidant by donating one or two electrons to a variety of oxidants, including oxygen free radicals and peroxides. Vitamin E is lipid-soluble and acts as a free radical scavenger in the membrane.205
Methemoglobin Formation and Reduction
About 3% of hemoglobin (Fe+2) is oxidized to methemoglobin (Fe+3) each day. Methemoglobin is unable to bind O2. To prevent hypoxemia, which would result from the accumulation of a high level of methemoglobin, the methemoglobin formed is reduced back to functional hemoglobin in a reaction that requires the cytochrome-b5 reductase (Cb5R) enzyme and NADH generated by anaerobic glycolysis.205 An inherited deficiency in Cb5R in dogs and cats results in persistent methemoglobinemia with minimal or no clinical signs.203 Methemoglobinemia also occurs in horses that have decreased Cb5R activity secondary to erythrocyte FAD deficiency.212
Iron Metabolism
Iron metabolism is presented in this chapter because more iron is needed for the production of erythrocytes than for all other cells in the body combined. Iron is absorbed from the diet in the small intestine and transferred to plasma, where it is bound to transferrin for transport to cells within the body. Once inside the body, iron cycles in a nearly closed system (Fig. 4-11) because little iron is lost in domestic animals unless hemorrhage occurs. About 75% of the iron present in plasma will be transported to the bone marrow for incorporation into hemoglobin in developing erythroid cells.436 The remaining plasma iron is taken up by nonerythroid tissues, primarily the liver.266 Erythrocytes containing hemoglobin normally circulate for several months before being phagocytized by macrophages when senescent. After phagocytosis, erythrocytes are lysed, hemoglobin is degraded, and iron is released. Most iron released from degraded hemoglobin is quickly released back into plasma, but a small amount may be stored as ferritin or hemosiderin within macrophages, which is released more slowly into plasma. The vast majority of iron entering plasma each day comes from macrophage release.204
About 60% to 70% of total body iron is present in hemoglobin (3.4 mg iron per gram of hemoglobin). About a third of total body iron is stored as ferritin and hemosiderin (primarily within macrophages), 3% to 7% is present in myoglobin (with the higher values occurring in dogs and horses), 1% is present in hemoprotein and flavoprotein enzymes, and only 0.1% is bound to transferrin in plasma.204
Iron Absorption
The absorption of iron from the diet depends upon age, species, iron stores, rate of erythropoiesis, inflammation, and pregnancy, as well as the amount and chemical form of iron ingested. A low percentage of dietary iron is absorbed in normal adult animals. Iron absorption occurs through enterocytes of the duodenum and proximal jejunum. Iron can be taken in by enterocytes as free ions or as heme by different pathways (Fig. 4-12). The relative importance of these pathways varies depending on species and diet.204
Most inorganic iron in the diet is in the ferric (Fe+3) state. Fe+3 iron is solubilized from food by hydrochloric acid in the stomach and binds to mucins and various small molecules in the stomach, which keep the iron soluble and available for absorption in the more alkaline environment of the small intestine. The most important pathway for nonheme iron uptake utilizes the divalent metal transporter-1 (DMT1). Fe+3 ions must be reduced to ferrous (Fe+2) ions before they can be transported into the enterocyte via the DMT1. Although some Fe+3 ion reduction may occur by direct interaction with dietary ascorbic acid, most reduction appears to rely on duodenal cytochrome b (DcytB) and possibly other brush border ferrireductase enzymes. Although humans absorb Fe+2 salts more readily from the intestine than Fe+3 salts, dogs are reported to absorb both valence forms equally well.204
Heme is released from dietary myoglobin and hemoglobin by the action of digestive enzymes. Dietary heme iron is generally more bioavailable than is nonheme iron and is an important nutritional source of iron in carnivores and omnivores. Heme enters duodenal enterocytes as an intact metalloporphyrin, possibly using a brush border transporter named heme carrier protein 1 (HCP1). However, this protein transports folate more efficiently than heme; consequently, its physiologic role in intestinal heme uptake remains to be clearly defined. After heme absorption, iron is released from heme by the action of the heme oxygenase reaction.20
Once within the enterocyte, intracellular iron molecules are likely bound to one or more chaperone molecules. A potential chaperone named poly (rC)-binding protein 1 (PCBP1) has been described.422 Iron taken up by enterocytes has one of two fates, export or storage, depending on the body’s iron needs. If iron is required by the body, molecules will be transported from enterocytes to transferrin in plasma. This transportation is mediated by ferroportin, an iron transport protein located on the basolateral surface of mature enterocytes. In addition to ferroportin, the efflux of iron from enterocytes requires a copper-containing protein called hephaestin, which is also located on the basolateral membranes of mature enterocytes. Hephaestin is a membrane-bound ferroxidase that has significant homology to the plasma protein ceruloplasmin. Hephaestin’s function may relate to its ability to oxidize Fe+2 ions to Fe+3 ions for binding to transferrin in plasma.20
If body iron requirements are low, enterocyte cytoplasmic iron accumulates. Free iron is toxic; consequently the mucosal cell protects itself by increasing apoferritin synthesis and incorporating the excess iron into ferritin. Each ferritin molecule is composed of a protein shell of 24 apoferritin subunits surrounding a central core of up to 4500 iron atoms as ferric oxyhydroxide. Ferritin is a storage protein that prevents free iron from catalyzing oxidative reactions, which would injure the cell. Ferritin within mucosal cells is returned to the small intestine lumen when enterocytes are sloughed at the tip of the villus after 1 to 2 days.445
Iron absorption is increased when total body iron content is low or erythropoiesis is increased. Iron absorption is decreased when total body iron content is high or inflammation is present.204 Components of brush-border iron uptake, including DMT1 and DcytB, are strongly influenced by the iron concentration within enterocytes, with increased components expressed when intracellular iron content is low and decreased components expressed when iron content is high. These locally responsive changes in brush-border transport components help buffer the body against the absorption of excessive iron, but it is the control of the basolateral transport of iron from enterocytes to plasma that represents the primary site at which iron absorption is regulated.445
Systemic Control of Iron Metabolism
Hepcidin, a peptide secreted by hepatocytes into the circulation, is an important systemic regulator of iron metabolism.153 Its production is modulated by body iron requirements, which are largely influenced by the magnitude of erythropoiesis present.354 Hepcidin inhibits iron export from enterocytes, macrophages, and hepatocytes by interacting directly with ferroportin, leading to the internalization and lysosomal degradation of this iron export protein.20 Hepcidin production is decreased in iron deficiency and disorders resulting in increased erythropoiesis, which increase the demand for iron.152 As a result, ferroportin receptors are abundant on cell surfaces and dietary iron absorption is increased, as is the export of iron from macrophages and hepatocytes. Conversely, hepcidin production is increased and ferroportin transporter expression on cell surfaces is decreased when iron overload is present. Hepcidin production is also increased during inflammation by a pathway not dependent on body iron requirements.445
Plasma Iron
Nearly all of the iron in plasma is bound to the protein apotransferrin to form transferrin. The binding of iron to apotransferrin keeps iron molecules soluble and prevents iron-catalyzed oxidative reactions. Apotransferrin is a β-globulin with two binding sites for Fe+3. Normally, 25% to 50% of the iron-binding sites are saturated with iron. Plasma iron turns over rapidly in 3 hours or less.436 Nearly all of the iron in plasma under normal conditions comes from the release of iron by macrophages that have phagocytized and degraded erythrocytes. In contrast, in normal individuals, only about 3% of the iron in plasma results from enterocyte absorption.372
Iron Uptake by Erythroid Cells
The delivery of iron from plasma to developing erythroid cells and other cell types except macrophages is dependent on transferrin.266 Transferrin (especially diferric transferrin) molecules bind to transferrin receptor 1 (TfR1) on the surface of cells, and these transferrin-TfR1 complexes invaginate to initiate endocytosis. After the transferrin-TfR1 complexes are internalized as endosomes, a proton pump decreases the pH in the endosome, resulting in conformational changes in the proteins and subsequent release of iron ions from transferrin. The released iron is exported from the endosome using DMT1.349 The resultant apotransferrin-TfR1 complex is recycled to the cell membrane, where apotransferrin is released from the cell, and the TfR1 is again available for binding additional iron-containing transferrin molecules. Erythroid precursor cells in the bone marrow and reticulocytes that synthesize hemoglobin have TfR1 on their surfaces for iron uptake, but reticulocytes lose their TfR1 as they develop into mature erythrocytes.372
After its release from transferrin, iron is transported to the mitochondria, where it is incorporated into protoporphyrin to form heme. A direct interorganelle transfer of iron occurs between endosomes and mitochondria in developing erythroid cells.419 Some iron is presumably released from endosomes into a cytoplasmic labile iron pool, with excess cytoplasmic iron stored as ferritin. TfR and apoferritin synthesis are regulated by the amount of intracellular iron present. High iron content stimulates apoferritin synthesis and inhibits TfR expression to minimize the potential of iron toxicity to the cell. Low iron content results in decreased apoferritin synthesis and increased TfR expression on cell surfaces to maximize iron uptake and use for heme synthesis. Free heme concentration within erythroid cells controls hemoglobin synthesis. An increase in free heme promotes the synthesis of globin chains and inhibits the uptake of iron from transferrin.204
Macrophage Iron Metabolism
Little iron enters macrophages, in contrast to other cell types in the body, via plasma transferrin. Rather, nearly all iron enters macrophages by the phagocytosis of aged or prematurely damaged erythrocytes (Fig. 4-13).373 Following phagocytosis, erythrocytes are lysed and hemoglobin is degraded to heme and globin. The microsomal heme oxygenase reaction degrades heme and releases iron. Most of the iron released from degraded heme is quickly exported from the macrophage and bound to plasma transferrin for transport to other cells (especially erythrocyte precursors in the bone marrow).204 The export of iron from macrophages is mediated by ferroportin and controlled by hepcidin, as has been discussed for enterocytes.495 The copper-containing plasma protein ceruloplasmin oxidizes Fe+2 ions to Fe+3 ions for binding to transferrin in plasma.341
The mononuclear phagocyte system accounts for much of the total body iron stores. Iron not rapidly released to plasma is stored within macrophages as ferritin and hemosiderin. Free cytoplasmic ferritin molecules are visible by electron microscopy but not by light microscopy. Hemosiderin is composed of aggregates of protein and iron within lysosomes. It is insoluble in water and thought to result from the degradation of ferritin. Hemosiderin is visible by light microscopy when it is stained with an iron stain (Prussian blue stain). Iron in the storage pool turns over slowly unless there is an increased need for iron for hemoglobin synthesis.45
Erythrocyte Destruction
Normal Removal of Aged Erythrocytes
Most erythrocytes circulate in blood for a finite time period (survival time or life span) ranging from 2 to 5 months in domestic animals, depending on the species. Erythrocyte life spans are related to body weight (and consequently metabolic rate), with the smallest animals (highest metabolic rate) having the shortest erythrocyte life spans. Greyhound dogs are often used as blood donors. The erythrocyte life span of 6 greyhound dogs (mean 93 days) was not significantly different from that of 3 nongreyhound dogs (103 days).158 Aged erythrocytes are phagocytized by macrophages of the mononuclear phagocyte system. Oxidative injury, especially near the end of their life span, appears to be responsible for normal erythrocyte aging and removal.205 Oxidative damage and other stressors can induce suicidal death of erythrocytes (eryptosis), with reactions similar to some of those that occur during apoptosis of nucleated cells. Eryptosis is characterized by Ca+2 entry, erythrocyte shrinkage, membrane blebbing (microvesicle formation), and cell membrane phospholipid scrambling, with phosphatidylserine exposure on the cell surface.271
Surface membrane alterations on aged or damaged cells that may be recognized by macrophages include exposure of phosphatidylserine on the external surface, modified external membrane carbohydrate residues (e.g., desialation of sialoglycoproteins), and/or modified membrane proteins (e.g., partially degraded band 3); these are possible signals for removal.57,246,265 Phosphatidylserine is normally localized in the inner leaflet of the lipid bilayer, but with cell damage phosphatidylserine may be exposed on the outer leaflet of the lipid bilayer, where it can be bound by phosphatidylserine receptors such as CD36 on the surface of macrophages.249 Other macrophage receptors can recognize altered carbohydrate moieties on the surface of erythrocytes.
The appearance of a senescent cell antigen may be an important signal in the removal of aged erythrocytes.246 This senescent cell antigen is derived from the band 3 anion transporter. The specific alteration required for band 3 to become antigenic remains to be clarified, but oxidative mechanisms are probably involved. A natural antibody against the senescent cell antigen is present in human plasma. This antibody binds to senescent cell antigens on the surface of aged cells and, together with bound complement, promotes the phagocytosis of aged erythrocytes by macrophages that exhibit Fc and C3b surface receptors. Senescent dog erythrocytes accumulate surface-associated immunoglobulin, which is believed to promote their removal by macrophages.395 The relative importance of the immune- and nonimmune-mediated phagocytosis of senescent erythrocytes remains to be clarified.
Erythrocytes lose volume by shedding microvesicles as they age. The composition of the resultant microvesicles varies, but they typically contain hemoglobin. Other components that may be present include glycophorin A, breakdown products of band 3 (senescent antigen), IgG, and exposed phosphatidylserine. They do not contain the skeletal proteins spectrin and ankyrin.186,536 Microvesicles are rapidly cleared from the circulation by macrophages, using receptors discussed above for aged erythrocytes.186 It may be that this process of microvesiculation removes patches of damaged membrane that would otherwise bind to macrophages and result in the early removal of otherwise healthy erythrocytes.536
Following phagocytosis by macrophages of the spleen, liver, and other organs, erythrocytes and erythrocyte microvesicles are lysed and hemoglobin is degraded to heme and globin (Fig. 4-14). Globin is catabolized to constituent amino acids, and the microsomal heme oxygenase reaction within macrophages degrades heme to iron, biliverdin, and carbon monoxide. Biliverdin is reduced to bilirubin via biliverdin reductase in nearly all mammals. Biliverdin reductase activity is low in rabbits and nutria and almost completely lacking in birds; consequently biliverdin is the predominant bile pigment in these species.101,476 Considerable heterogeneity exists in reptiles, amphibians, and fish in the production of bilirubin versus biliverdin.101 Bilirubin is released from macrophages and bound to albumin for transport to the liver for conjugation and excretion. Approximately 80% of the bilirubin produced in the body comes from the degradation of hemoglobin, with the remainder coming from the degradation of other heme-containing proteins.476
Pathologic Destruction of Erythrocytes
Increased eryptosis (similar to the apoptosis of nucleated cells) may contribute to the development of anemia in some disorders. As discussed earlier, eryptosis is characterized by Ca+2 entry, erythrocyte shrinkage, and membrane blebbing with ectosome formation (Fig. 4-15).271 Reported triggers of eryptosis include oxidative stress, energy depletion, osmotic shock, lipid-derived signaling molecules, certain bacterial exotoxins, various drugs, and metals including lead, copper, zinc, and mercury. Diseases associated with accelerated eryptosis in humans include sepsis, malaria, hemoglobinopathies, G6PD deficiency, phosphate depletion, iron deficiency, and hemolytic uremic syndrome.270
Almost no lysis of erythrocytes occurs within the circulation of normal individuals, but intravascular hemolysis can be present when severe membrane damage occurs in disease states (Fig. 4-16). Following lysis, hemoglobin in plasma (hemoglobinemia) reversibly dissociates into dimers that bind almost irreversibly to haptoglobin, an α2-glycoprotein in plasma. The hemoglobin-haptoglobin complex is too large to be filtered through the kidney and is rapidly removed from the circulation following binding to the hemoglobin scavenger receptor CD163 on macrophages. Once inside the cell, hemoglobin-haptoglobin complexes are transported to lysosomes for degradation, and receptors are recycled to the cell surface.229 The hemoglobin is degraded and iron is conserved, as discussed previously.
Once plasma haptoglobin is saturated (about 50 to 150 mg/dL of hemoglobin-binding capacity in dogs, cats, and horses), remaining free hemoglobin dimers are small enough to be readily filtered by the kidney.196 Some hemoglobin is reabsorbed by the proximal tubules, but once that capacity is exceeded, hemoglobin appears in the urine (hemoglobinuria).211 Plasma appears red when as little as 50 mg/dL of hemoglobin is present; consequently hemoglobinemia may be observed in the absence of hemoglobinuria. Hemoglobin absorbed by the proximal tubules is rapidly catabolized, and iron is stored as ferritin and hemosiderin.203 Iron that is not reutilized is lost when tubular epithelial cells slough into the urine.
Free hemoglobin in plasma can spontaneously oxidize to form methemoglobin, which tends to dissociate into ferriheme (hemin) and globin. Free heme binds to a plasma protein called hemopexin. The heme-hemopexin complexes undergo endocytosis after binding to CD91 on the surface of macrophages and hepatocytes.229 The binding to hemopexin protects cell membranes from toxic effects of free heme, and it also conserves iron. Albumin from primates can also bind heme to form methemalbumin, but albumin from common domestic animals does not bind heme.163
Abnormal Erythrocyte Morphology
Rouleaux
Erythrocytes on blood films from healthy horses, cats, and pigs often exhibit rouleaux (aggregations of erythrocytes grouped together like a stack of coins) formations (see Fig. 4-2). Rouleaux formation depends on both the nature of the erythrocytes and the composition of plasma.37 Erythrocytes that are more deformable and have greater membrane fluidity with less negative charge on their surfaces (weaker electrostatic repulsive force) aggregate more readily than cells with the opposite characteristics.37,443,460 Rouleaux formation also depends on the presence of high-molecular-weight proteins in plasma.132 Increased concentrations of globulin proteins—including fibrinogen, haptoglobin, and immunoglobulins—potentiate rouleaux formation in association with inflammatory conditions.11,532 Rouleaux formation can also occur in association with some lymphoproliferative disorders in which one or more immunoglobulins are secreted in high amounts (Fig. 4-17). Prominent rouleaux formation in species other than horses, cats, or pigs should be noted as an abnormal finding.
Agglutination
Aggregation or clumping of erythrocytes in clusters (not in chains, as in rouleaux) is termed agglutination (Fig. 4-18). Agglutination is caused by the occurrence of immunoglobulins bound to erythrocyte surfaces. Because of their pentavalent nature, IgM immunoglobulins have the greatest propensity to produce agglutination.129 EDTA-dependent IgM-mediated erythrocyte agglutination has been reported in a cat without evidence of hemolysis. Agglutination did not occur if blood was collected in heparin or citrate.411 High-dose unfractionated heparin treatment in horses also causes erythrocyte agglutination by an undefined mechanism.319,322
Polychromasia
The presence of bluish-red erythrocytes in stained blood films is called polychromasia (Fig. 4-19). Polychromatophilic erythrocytes are reticulocytes that stain bluish-red owing to the combined presence of hemoglobin (red staining) and individual ribosomes and polyribosomes (blue staining). Low numbers of polychromatophilic erythrocytes are usually seen in blood from normal dogs and pigs, because up to 1.5% reticulocytes may be present in dogs and up to 1% reticulocytes may be present in pigs even when the HCT is normal.238 Slight polychromasia may be present in normal cats, but many normal cats exhibit no polychromasia in stained blood films. Polychromasia is absent in stained blood films from normal cattle, sheep, goats, and horses because reticulocytes with sufficient RNA to impart a bluish color are not normally present in the blood in these species.
The most useful approach in the classification of anemia is to determine whether or not evidence of a bone marrow response to the anemia is present in blood. For all species except the horse, this involves determining whether absolute reticulocyte numbers are increased in blood. Horses rarely release reticulocytes from the bone marrow even when an increased production of erythrocytes occurs. When an absolute reticulocytosis is present, the animal is said to have a regenerative anemia. The presence of a regenerative response suggests that the anemia results from either increased erythrocyte destruction or hemorrhage. A nonregenerative anemia generally indicates that the anemia is the result of decreased erythrocyte production (Fig. 4-20); however, about 3 to 5 days are required for increased reticulocyte production and release by the bone marrow in response to an acute anemia.15,65,146,364 Consequently the anemia appears nonregenerative shortly after hemolysis or hemorrhage has occurred (see Fig. 4-4).
Increased polychromasia is usually present in regenerative anemias because many reticulocytes stain bluish-red with routine blood stains (see Fig. 4-19). When the degree of anemia is severe, basophilic macroreticulocytes or so-called stress reticulocytes or shift reticulocytes may be released into the blood (Fig. 4-21). High concentrations of erythropoietin shorten the marrow transit time for erythroid cells, resulting in the early release of immature reticulocytes that are twice the normal size.379 There is a direct correlation between the percentage of polychromatophilic erythrocytes and the percentage of reticulocytes in dogs (and presumably in pigs) and between the percentage of polychromatophilic erythrocytes and percentage of aggregate reticulocytes in cats (Fig. 4-22).15,269 Cats with mild anemia may not release aggregate reticulocytes from the marrow but will release punctate reticulocytes (Fig. 4-23, A).15 Because punctate reticulocytes do not contain sufficient numbers of ribosomes to impart a bluish color to the cytoplasm, mild regenerative anemia in cats may lack polychromasia in stained blood films (Fig. 4-23, B).
Anisocytosis
Variation in erythrocyte diameters in stained blood films is called anisocytosis (see Fig. 4-23, B). It is greater in normal cattle than in other normal domestic animals.238 Anisocytosis is increased when different populations of cells are present, as can occur following a transfusion (Fig. 4-24). Anisocytosis may occur when substantial numbers of smaller than normal cells are produced, as occurs with iron deficiency, or when substantial numbers of larger than normal cells are produced, as occurs when increased numbers of reticulocytes are produced. Consequently increased anisocytosis is usually present in regenerative anemia (see Figs. 4-18, 4-19, 4-21), but it may be present in some cases of nonregenerative anemia resulting from dyserythropoiesis.199 Anisocytosis without polychromasia may be seen in horses with intensely regenerative anemia (Fig. 4-25).
Hypochromasia
The presence of erythrocytes with decreased hemoglobin concentration and increased central pallor is called hypochromasia (Figs. 4-26 through 4-32). Not only is the center of the cell paler than normal but the diameter of the area of central pallor is increased relative to the red-staining periphery of the cell. True hypochromic erythrocytes must be differentiated from torocytes, which have colorless punched-out centers but wider dense red-staining peripheries (Fig. 4-33).43,238 Torocytes are generally artifacts. Increased hypochromasia is observed in iron-deficiency anemia.
Erythrocytes from dogs, ruminants, and pigs with iron-deficiency anemia often appear hypochromic on stained blood smears. Hypochromasia is less prominent (see Fig. 4-24) and generally not recognized in iron-deficient cats and horses. Hypochromasia in iron deficiency results from both decreased hemoglobin concentration within cells and from the fact that the cells are thin leptocytes (Fig. 4-34, A). Because these microcytic leptocytes have increased diameter-to-volume ratios, they may not appear as small cells when viewed in stained blood films (Fig. 4-34, B).210 Microcytic erythrocytes from iron-deficient llamas and alpacas exhibit irregular or eccentric areas of hypochromasia within the cells (see Figs. 4-31, 4-32).326
Poikilocytosis
Erythrocytes can assume a wide variety of shapes. Poikilocytosis is a general term used to describe the presence of abnormally shaped erythrocytes. Although specific terminology is used for certain abnormal shapes, it is less important to quantify each type of shape change than it is to determine the cause of the shape change.509 Poikilocytosis may be present in clinically normal goats and young cattle (Figs. 4-5, 4-35).225,409 In some instances, these shapes appear to be related to the hemoglobin types present, but an abnormality in protein 4.2 in the membrane has been suggested as a causative factor in calves.352 Increased numbers of poikilocytes are also present in human preterm and term neonates.403
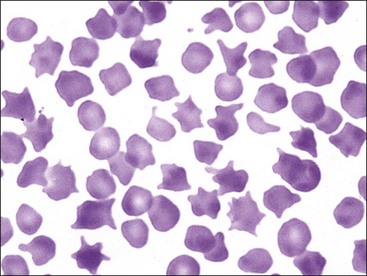
FIGURE 4-35 Poikilocytosis (acanthocytes and echinocytes) in blood from a nonanemic young calf. Wright-Giemsa stain.
Poikilocytosis forms in various disorders associated with erythrocyte fragmentation, including disseminated intravascular coagulation (DIC), liver disease, myeloid neoplasms, myelofibrosis, glomerulonephritis, and hemangiosarcoma (dogs).392 For unknown reasons, severe iron-deficiency anemia in dogs, pigs, and ruminants may exhibit pronounced poikilocytosis (see Figs. 4-27 through 4-32).201 Poikilocytes can form when oxidant injury results in Heinz body formation and/or membrane injury. One or more blunt erythrocyte surface projections may form as the membrane adheres to Heinz bodies bound to its internal surface.392 Various abnormalities in erythrocyte shape were reported in horses with intravascular hemolysis resulting from severe cutaneous burn injuries. Membrane blebbing with fragmentation was prominent (Fig. 4-36).347 A variety of abnormal erythrocyte shapes have been reported in dogs and cats with doxorubicin toxicity32,348 and in dogs with dyserythropoiesis.223
Echinocytes (Crenated Erythrocytes)
Echinocytes are spiculated erythrocytes in which the spicules are relatively evenly spaced and of similar size.523 Spicules may be sharp or blunt. When observed in stained blood films, echinocytosis is usually an artifact that results from excess EDTA, improper smear preparation, or prolonged sample storage before blood film preparation. The appearance of the echinocytes can vary depending on the thickness of the blood film (Fig. 4-37). They are common in normal pig blood smears (Fig. 4-38), forming in vitro.238 The morphology of echinocytes varies from slightly spiculated echinodiscocytes to highly spiculated spheroechinocytes, which have been called burr cells (Fig. 4-39, A). The most advanced echinocytes are those that have lost most of their spicules and have nearly become spherocytes (Fig. 4-39, B). Echinocytes form when the surface area of the outer lipid monolayer increases relative to the inner monolayer.438
Echinocytic transformation occurs in the presence of fatty acids, lysophospholipids, and amphiphatic drugs that distribute preferentially in the outer half of the lipid bilayer.205 Transient echinocytosis occurs in horses with Clostridium perfringens infection526 and in dogs following rattlesnake (see Fig. 4-39, A),70,191 coral snake (see Fig. 4-39, B),301 water moccasin (Fig. 4-40), and asp viper (Vipera aspis) envenomation,303 presumably secondary to the action of phospholipases present in venom.498 Depending on the time course and dose of venom received, either echinocytosis or spherocytosis may be observed after these snakebites.
Echinocytes may occur in uremic animals and immediately after transfusion of stored blood.392 They have been seen with increased frequency in dogs with glomerulonephritis and neoplasia (lymphoma, hemangiosarcoma, mast cell tumor, and carcinoma).391,523 Echinocytes are the predominant erythrocyte shape abnormality in human burn patients, and this was one of the shape abnormalities recognized in horses with severe cutaneous burn injuries (see Fig. 4-36).195,347
Echinocytes also form when erythrocytes are dehydrated, pH is increased, ATP is depleted, and intracellular calcium is increased.31,205 Echinocytosis occurs in horses when total body depletion of cations has occurred (endurance exercise, furosemide treatment, diarrhea, systemic disease).162,205
Although the mechanisms are not fully understood, ATP is required for the maintenance of normal shape and deformability of erythrocytes.205 Phospholipids are asymmetrically arranged in the plasma membrane, with anionic amino-containing phospholipids (predominantly phosphatidylserine) located in the inner leaflet of the bilaminar membrane. These anionic phospholipids are shuttled (flipped) from the outer leaflet to the inner leaflet by an ATP-dependent aminophospholipid-specific translocase or flippase.553 ATP also provides the energy needed to pump Ca+2 out of cells. Increased Ca+2 activates neutral proteases (calpains), which can degrade membrane skeletal proteins; phospholipase C, which cleaves phosphoinositides; and scramblase, which accelerates the bidirectional transbilayer movement of phospholipids.205,540 The inhibition of the flippase and/or the activation of the scramblase can result in increased phosphatidylserine in the outer layer, which appears to promote echinocyte formation, as well as coagulation and erythrophagocytosis.116,295,406
Echinocytes and other shape abnormalities have been recognized in dogs with erythrocyte pyruvate kinase deficiency (Fig. 4-41), which results in a decreased ability to generate ATP. 86,328,412 Occasional echinocytes may also be seen in dogs with erythrocyte phosphofructokinase deficiency (Fig. 4-42). PFK-deficient erythrocytes have decreased ATP and increased Ca+2 concentrations.404
Acanthocytes
Erythrocytes with irregularly spaced, variably sized spicules are called acanthocytes or spur cells (Fig. 4-43).43 Acanthocytes form when erythrocyte membranes contain excess cholesterol compared to phospholipids. If cholesterol and phospholipids are increased to a similar degree, codocyte formation is more likely than acanthocyte formation.98 Alterations in erythrocyte membrane lipids can result from increased blood cholesterol content or due to the presence of abnormal plasma lipoprotein composition.99 Acanthocytes have been recognized in animals with liver disease (Fig. 4-44), possibly due to alterations in plasma lipid composition, which can alter erythrocyte lipid composition.91,392,522 They have also been reported in dogs with disorders that result in erythrocyte fragmentation, such as hemangiosarcoma (Fig. 4-45), DIC, and glomerulonephritis.342,471,522
Marked acanthocytosis is reported to occur in young goats and some young cattle (see Fig. 4-35). Acanthocytosis of young goats occurs as a result of the presence of hemoglobin C at this early stage of development.205
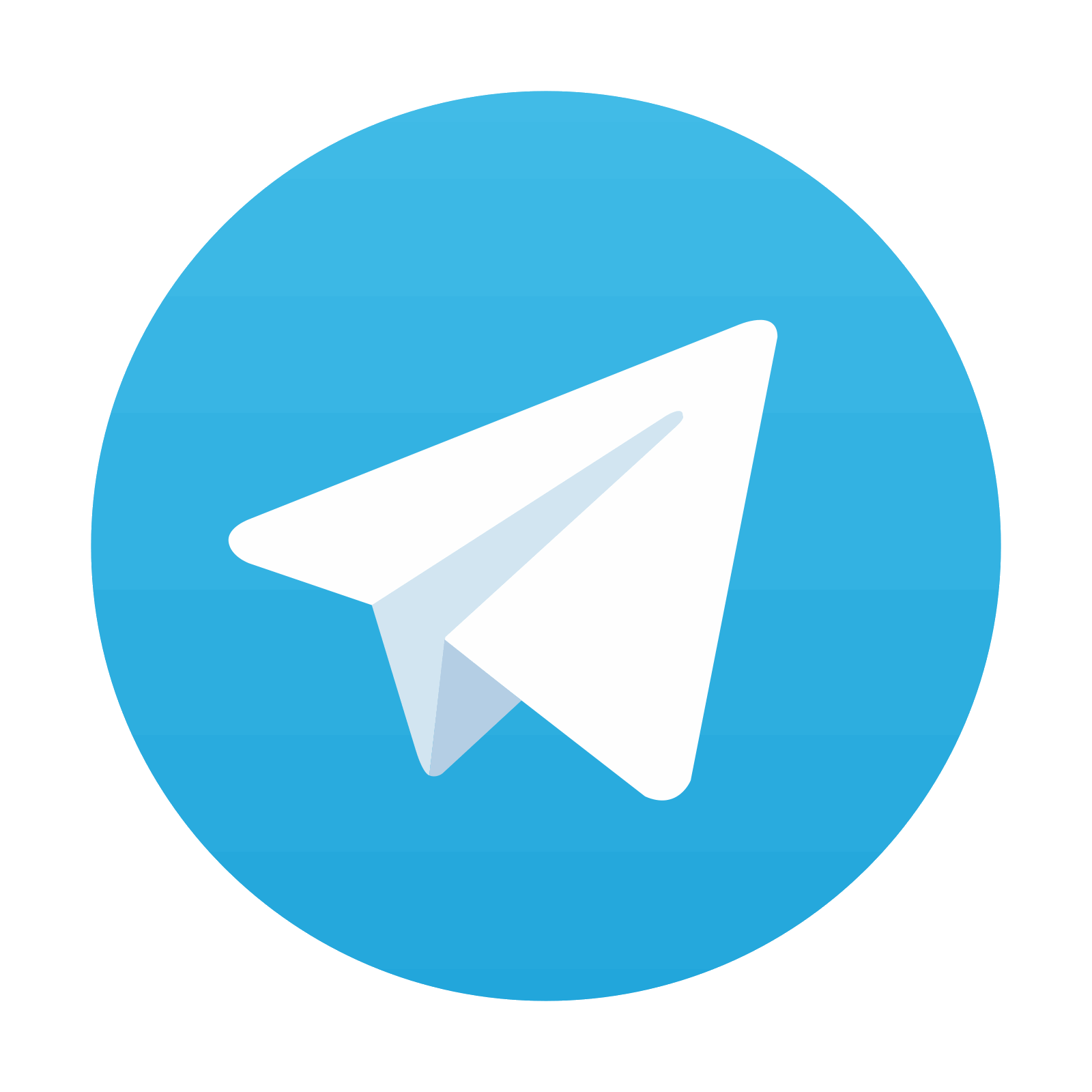
Stay updated, free articles. Join our Telegram channel
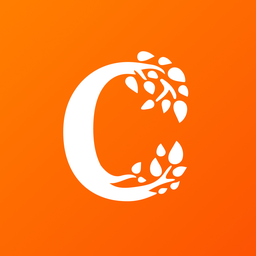
Full access? Get Clinical Tree
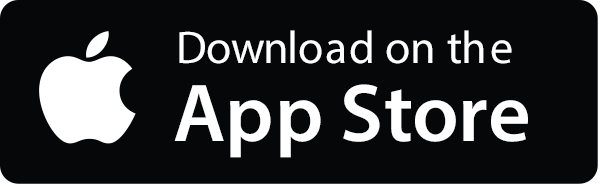
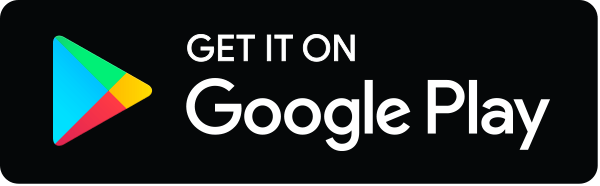