CHAPTER 7 Curtis W. Dewey This chapter focuses on brain diseases other than head trauma and cerebellar disorders. These latter subjects are discussed in detail in Chapters 8 and 12, respectively. There are a large number of diseases that can affect the brain, the majority of which are discussed in this chapter. Seizures will be mentioned where appropriate, but the important subject of seizure disorders and their management is discussed in detail in Chapter 9. Many of the disease processes that affect the brain can cause dramatic clinical signs, which can be very upsetting to the owner and even the clinician. However, many of these diseases can be successfully treated. The clinician should be cautious not to rush into making prognostic decisions based primarily on clinical appearance. Cerebrum/diencephalon (forebrain) Dogs and cats with forebrain dysfunction may exhibit clinical signs that include altered mental status (obtundation more likely than stupor or coma), behavioral changes, circling in a wide arc, head-pressing, visual impairment, focal and/or generalized seizure activity, and hemi-inattention (unilateral hemineglect) syndrome. The combination of conscious proprioceptive deficits with a normal to near-normal gait is characteristic of forebrain dysfunction. Neck pain may be appreciable in patients with structural brain lesions. Brain stem (caudal to the diencephalon) Lesions from the midbrain (mesencephalon) through the medulla myelencephalon) may lead to altered mental status (stupor or coma more likely than with forebrain lesions), proprioceptive and gait abnormalities, deficits in s (CN) III–XII, and central vestibular dysfunction. Table 7.1 Encephalopathies of dogs and cats. Table 7.2 Lysosomal storage diseases of dogs and cats. a Puppies display generalized seizures, obtundation, tremors, and weakness associated with hypoglycemia. b Exhibited features of type I and type II disease. c These cats have an increased risk for developing intracranial meningioma.230 d Specific enzyme and/or genetic defect has been identified for this breed. e Exhibited adult onset degeneration of cerebellar and thalamic nuclei with lipopigment accumulation in affected cells.529 For most of these diseases, affected animals are normal at birth, and develop a progressive multifocal to diffuse encephalopathy within the first several weeks to several months of life. Many of the storage disorders have the common feature of cerebellar dysfunction as an early sign of disease. Examples include mannosidosis, the gangliosidoses (GM1, GM2), globoid cell leukodystrophy (Krabbe’s disease), glucocerebrosidosis (Gaucher’s disease), and sphingomyelinosis (Niemann–Pick disease). Forebrain dysfunction predominates, at least in the disease’s early stages, in neuronal glycoproteinosis (Lafora’s disease), ceroid lipofuscinosis (Batten’s disease), and fucosidosis. Dogs with Lafora’s disease typically develop clinical signs of dysfunction (seizures, dementia) within the first year of life (Video 14). Ceroid lipofuscinosis and fucosidosis are unique among the lysosomal storage disorders in their relatively late onset of clinical signs. Although clinical signs of neurologic dysfunction occasionally occur in dogs less than 1 yr of age for these two diseases, disease onset is typically in young adult dogs. Encephalopathic signs of ceroid lipofuscinosis initially manifest at 1–2 yrs of age, although the age range of disease onset is 6 mos to 10 yrs. Behavior changes and visual deficits are usually the first abnormalities noticed. The disease typically progresses over one to several years to include neurologic abnormalities such as seizures, ataxia, tremors, and hypermetric gait. Two cats that were reported with ceroid lipofuscinosis exhibited rapid deterioration of neurologic status. A form of ceroid lipofuscinosis that selectively involves neurons in the cerebellum and thalamus of adult dogs has been described. These dogs exhibited signs of progressive cerebellar dysfunction. Although the age range for onset of neurologic dysfunction in fucosidosis is 4–24 mos, most dogs begin to exhibit signs of an abnormality between 12 and 18 mos of age. Forebrain dysfunction (e.g. behavior change, circling) is evident initially, and progresses over 2–3 yrs to include signs such as ataxia, dysphagia, vision and hearing loss, nystagmus, and dysphonia. Enlargement of the ulnar nerves is often palpable in dogs with fucosidosis. The enlargement is due to both edema and infiltration of the nerves with lipid-filled phagocytes and Schwann cells. Involvement of areas other than the brain is a feature of the lysosomal storage diseases that may affect the clinical manifestation of a particular disease, the accessibility of tissue for diagnostic purposes, or both. Organomegaly (e.g. hepatomegaly, splenomegaly) is apparent upon physical examination for some of these diseases, due to accumulation of storage products in cells of the abdominal organs. Skeletal abnormalities (e.g. craniofacial malformation, joint immobility) are a common feature of mannosidosis, the mucopolysaccharidoses, and mucolipidosis II. Pelvic limb paresis often develops in cases of mucopolysaccharidosis and mucolipidosis, due to impingement of the spinal cord by bony vertebral growths invading the vertebral canal. Skeletal abnormalities and widened intervertebral disc spaces may also occur in gangliosidosis of English Springer Spaniels and Portuguese Water dogs. Dwarfism associated with gangliosidosis has been described in English Springer Spaniels. Involvement of the PNS may occur with fucosidosis, globoid cell leukodystrophy, the glycogenoses, and sphingomyelinosis (Niemann–Pick disease). Several genes necessary for normal myelin assembly have been shown to be down regulated in dogs afflicted with fucosidosis. Myopathy is a prominent feature of the glycogenoses, but forebrain dysfunction may result from secondary hypoglycemia in some of these disorders. Corneal abnormalities have been observed in both gangliosidosis and mannosidosis of cats, and mucopolysaccharidosis type VII in dogs. Retinal degeneration has been reported in some dog breeds with ceroid lipofuscinosis (e.g. Tibetan Terrier, Miniature Schnauzer, Cocker Spaniel), and in cats with mucolipidosis II. Cardiac abnormalities can occur with the glycogenoses. Tentative diagnosis of a lysosomal storage disease is based upon clinical signs of a progressive, multifocal/diffuse encephalopathy in a young animal, especially in a susceptible breed. Cerebrospinal fluid (CSF) analysis is usually normal but may reveal increased protein levels with a normal cell count. Abnormalities of the brain may be evident on computed tomography (CT) or magnetic resonance imaging (MRI; e.g. ventriculomegaly, brain atrophy, abnormal brain tissue density). In one study of canine gangliosidosis (GM1) in which sequential brain MR imaging was performed, early evidence (by 2 mos of age) of diffuse cerebral white matter hyperintensity on T2-weighted (T2-W) and FLAIR images was found, in addition to brain atrophy later (around 9 mos of age) in the disease course (Fig. 7.1). Definitive diagnosis of a specific lysosomal storage disease is typically made by the identification of the storage product (antemortem or postmortem; Fig. 7.2), documenting the deficient enzyme activity, and/or demonstrating the presence of the defective gene responsible for the disease. Whole blood leukocytes, tissue biopsy samples (e.g. liver), or cultured fibroblasts can be used to demonstrate storage material and assay for deficient lysosomal enzyme activity. In some of the storage diseases (e.g. mannosidosis, mucopolysaccharidosis, fucosidosis), accumulated storage product can be identified in urine using specific assays. For many of the lysosomal storage diseases identified in veterinary patients, the specific genetic mutations responsible for the enzyme deficiencies have been identified and sequenced. It is now possible to diagnose some of the lysosomal storage diseases in dogs and cats by demonstrating the defective gene using a blood sample. Figure 7.1 Transaxial T2-weighted (A) and FLAIR (B) brain images of a dog with gangliosidosis (GM1) demonstrating diffuse cerebral white matter hyperintensity. (Hasegawa et al., 2012.)279 Figure 7.2 Brain histopathology of a cat with mannosidosis. Neurons swollen with storage product are evident. (Dr. Charles Vite, 2014. Reproduced with permission from Dr. Charles Vite.) Treatment of lysosomal storage diseases is directed at reducing the accumulation of cellular storage products. Various methods are used in people to increase the specific deficient enzyme activity in order to achieve this effect. They include bone marrow transplantation (transplanting stem cells that produce the deficient enzyme), enzyme replacement therapy (recombinant enzyme administered parenterally to the patient), and gene therapy (transferring normal copies of the malfunctioning gene to the patient’s cells for the specific disease using a viral vector). It has been shown that, in general, only about 5% of normal enzyme activity is necessary to prevent or reverse clinical signs associated with lysosomal storage diseases. Substrate reduction therapy (administering drugs that inhibit enzymes that produce the accumulated product) is a method to reduce the amount of substrate that should normally be degraded by the deficient enzyme. All of these options are associated with problems such as autoimmune responses to a transplanted foreign material, transient duration of effect, and inability to cross the blood–brain barrier. Despite these challenges, several successful interventions have been reported in dogs and cats with lysosomal storage diseases. Intravenous and/or intrathecal recombinant enzyme replacement therapy has been successfully used to treat canine fucosidosis, mucopolysaccharidosis type I, mucopolysaccharidosis type IIIA, and feline mucopolysaccharidosis type VI. Another promising therapeutic approach is the delivery of functional copies of the specific defective gene directly to the patient’s brain (either intrathecally or intraparenchymally) using a viral vector. The functional copy of the gene becomes incorporated into the target cell, which then begins to produce the deficient enzyme (cell transduction). This therapeutic approach has been shown to be effective in the treatment of feline α-mannosidosis and canine mucopolysaccharidosis types I and IIIB. In one study, the oral treatment of cats afflicted by Niemann–Pick disease type C with an imino sugar called miglusat resulted in the delayed onset of neurologic signs and increased lifespan, as well as decreased accumulation of gangliosides (GM2) in neurons and prolonged Purkinje cell survival. At present, the prognosis for lysosomal storage diseases remains guarded to poor. For many of these disorders, affected animals are euthanized due to progressively worsening neurologic dysfunction within the first year of life. For the more slowly progressive disorders (e.g. fucosidosis, ceroid lipofuscinosis), continuous neurologic dysfunction leads to death or euthanasia usually within 1–2 yrs of diagnosis. However, continued advances in molecular diagnosis as well as enzyme replacement and gene therapy for these disorders will likely lead to a more favorable prognosis for many lysosomal storage diseases in the near future. An age-related syndrome similar to Alzheimer’s disease (AD) in people occurs in elderly dogs and cats. Cognitive dysfunction syndrome is best described for the dog, and this species appears to be the best animal model available for human AD. Similar to AD of people, the pathophysiology of CDS is uncertain. There are pathologic similarities between the brains of humans with AD and dogs and cats with CDS. Cerebral vascular changes, meningeal thickening, gliosis, and ventricular dilatation occur in the brains of both AD and CDS patients. More specifically, the progressive accumulation of a neurotoxic protein called beta-amyloid in the brain (in and around neurons) is a consistent feature in both AD and CDS. These accumulations coalesce to form plaques (neuritic plaques) and are most prominent in the frontal cerebral cortex and in the hippocampus in both human and veterinary disorders. In both disorders, the degree of beta-amyloid accumulation correlates with the extent of cognitive impairment. In addition to the accumulation of neurotoxic beta-amyloid (Aβ) protein in the aged canine and feline brain, intraneuronal accumulation of a hyperphosphorylated microtubular-associated protein (tau protein) has also been demonstrated. Tau protein is the precursor to neurofibrillary tangles (NFTs), another prominent histopathologic feature of human AD. The absence of mature NFTs in the brains of dogs and cats with CDS has been argued as evidence against CDS of dogs and cats being analogous to human AD. However, the absence of NFTs in dogs and cats has a number of potential explanations. It is possible that dogs and cats do not live long enough for the tau proteins to develop into NFTs as they do in people. While the amino acid sequence of Aβ protein is identical between humans and dogs, this is not the case for tau protein. The amino acid sequence of dogs and cats differs from that in people; this different sequence may affect the ability of tau protein to form NFTs. Other structural abnormalities found in the aging canine brain that are similar to those in humans include cerebral atrophy, ventricular enlargement, blood vessel wall fibrosis, and amyloid deposition (meningeal and parenchymal), microhemorrhages and infarcts, axonal degeneration with myelin loss, astroglial hypertrophy and hyperplasia, and intraneuronal accumulation of several substances (lipofuscin, polyglucosan bodies, ubiquitin). The pathophysiology of CDS and AD is multifactorial and complex. There is evidence in both diseases that increased oxygen free radical mediated cellular damage, decreased endogenous antioxidant defenses, inflammation (from various processes), decreased mitochondrial function, DNA damage, altered gene expression, vascular compromise, decreased capacity for neurogenesis (likely associated with hippocampal degeneration), synaptic dysfunction, and neurotransmitter imbalance are all interrelated processes that are involved in progressive cognitive impairment. There is some evidence in people with AD that neurovascular damage precedes the accumulation of Aβ protein. Since brain hypoxia can stimulate the production of Aβ protein (by altering amyloid metabolism enzymes) and accumulated Aβ protein around brain blood vessels leads to progressive vascular damage, it is clear that a self-perpetuating cycle of vascularly mediated brain damage occurs in AD and CDS. Neurochemical changes that occur in the aging brain are thought to contribute to progressive cognitive impairment. An age-associated decline in the brain’s neurotransmitter levels of acetylcholine, dopamine, norepinephrine, serotonin, and gamma-aminobutyric acid (GABA) have been documented in CDS and AD. Other neurochemical abnormalities identified in the brains of CDS and AD patients include increased acetylcholinesterase levels (associated with cholinergic decline), increased monoamine oxidase B (catalyzes the breakdown of dopamine, with a subsequent formation of free radicals), and elevated CSF levels of lactate, pyruvate, and potassium. Cognitive dysfunction syndrome is recognized primarily in elderly dogs (more than 9 yrs) and cats (more than 12 yrs), but should be suspected in animals 7 yrs or older that are demonstrating progressive cognitive impairment. It is likely that many pets with mild cognitive impairment are not reported as such and that the majority of CDS patients seen by veterinarians are severely impaired, similar to human AD patients. Clinical signs of CDS are numerous and often nonspecific. They include inattentiveness, inactivity, aimless wandering (often pacing at night), walking in circles, demented behavior, disturbance of the sleep/wake cycle, urinary and/or fecal incontinence, difficulty navigating stairs, becoming lost in previously familiar environments, attempting to pass through narrow spaces (Fig. 7.3), failure to recognize previously familiar people or animals, decreased interaction with family members, hearing loss, and excessive vocalization (often at night). Cats with CDS occasionally exhibit overresponsive and aggressive behavioral patterns as well as excessive vocalization. The acronym DISHAAL (disorientation; alterations in interactions with owners, other pets, and the environment; sleep/wake cycle disturbances; house soiling; changes in activity; perceived anxiety; and learning or memory deficits) can be used as a checklist for patients suspected of being affected by CDS (Table 7.3). Owners of CDS pets often describe their pets as acting “senile.” Aging dogs appear to fall into three categories of cognitive function, similar to people. These categories are successful aging, mild cognitive impairment, and severe cognitive impairment (dementia). The third, most severe category is consistent with a diagnosis of AD. Standardized cognitive testing of older dogs (almost exclusively done in a research setting) has enabled a more objective determination of the extent of cognitive dysfunction in individuals, as well as the response to therapeutic interventions. Such testing includes the delayed nonmatching to position (DNMP) memory task (Fig. 7.4) and the attention task (Fig. 7.5). In addition to the classic behavioral abnormalities indicative of CDS in dogs, the author occasionally encounters suspected CDS dogs with either transient central vestibular dysfunction or seizure activity of recent onset. Although not yet reported as a clinical feature of canine CDS, vestibulocerebellar dysfunction and seizures are reported as a potential consequence of AD in people. Seizure activity has been reported associated with feline CDS. Figure 7.3 A dog with cognitive dysfunction syndrome (CDS) attempting to pass through the wrong end of the door. (Rofina et al., 2006. Reproduced with permission from Elsevier.)586 Figure 7.4 The DNMP is a test of short-term visuospatial working memory. The test consists of two phases. In the sample phase, the subject is required to displace an object placed over one of three possible locations on a food well (top); in this case the cat is required to displace block S covering food reward in the well on cat’s right. The second stage (bottom) occurs after a delay and the subject is presented with two objects identical to that used in the sample phase. One object (marked with an X) is located in the same position as the sample object. The correct object is located in one of the remaining two positions (the nonmatch), and if the subject displaces the object, it can retrieve the food reward beneath. Initially, subjects are trained using a 5-second delay between the phases, but when the cat learns the rule that the food will always be found under the block in the nonmatch position, gradually longer delays can be introduced to assess memory. (Landsberg et al., 2012. Reproduced with permission from Elsevier.)394 Figure 7.5 In the attention task, the dog must select the correct object (covering a food reward), which is presented concurrently with either one, two, or three incorrect objects (distracters). Studies have demonstrated that performance declines and latency increases with increased distracter number, consistent with a test that assesses selective attention. (Landsberg et al., 2012. Reproduced with permission from Elsevier.)394 Table 7.3 CDS checklist.1 a Score: 0 = none; 1 = mild; 2 = moderate; 3 = severe. Source: Adapted from Landsberg GM, Hunthausen W, Ackerman L. The Effects of Aging on the Behavior of Senior Pets: Handbook of behavior problems of the dog and cat. 2nd ed. Philadelphia: W.B. Saunders; 2003:273; with permission. Similar to AD of people, a diagnosis of CDS in a dog or cat is based primarily on historical complaints indicative of progressive cognitive impairment. Before arriving at a presumptive diagnosis of CDS, the clinician should rule out other potential causes of cognitive dysfunction, such as metabolic disorders (e.g. hepatic encephalopathy) and structural brain disorders (e.g. brain tumor). In AD, CT or MR imaging of the brain is usually performed as part of the diagnostic workup, and should ideally be part of the diagnostic plan for CDS patients. The brain imaging of AD patients can be normal, but may reveal brain atrophy, ventricular enlargement, and lesions in the medial temporal lobes of the cerebral cortex (Fig. 7.6). Age-related changes appreciated on MR imaging of the brain in CDS patients are primarily reflective of brain atrophy and include ventricular enlargement, widened and well-demarcated cerebral sulci, and diffuse and scattered areas of T2 hyperintensity, in periventricular white matter. Although these are consistent findings associated with the aging brain, they may be found in older patients without evidence of CDS. In one study, the thickness of the interthalamic adhesion as measured on transaxial T1- and T2-W MR images was found to be significantly smaller in dogs with CDS compared with dogs without CDS (Fig. 7.7). An interthalamic adhesion thickness of 5.0 mm or less was found to be consistent with a diagnosis of CDS in dogs. Positron emission tomography (PET) scanning with radioactively labeled glucose (to evaluate brain glucose utilization) is often used in the evaluation of human AD; this has been investigated as a potential tool for evaluating brain function in dogs. Figure 7.6 Transaxial MR image of a dog with cognitive dysfunction, demonstrating typical characteristics of brain aging. Figure 7.7 Transaxial (A) and midsagittal (B) T2-weighted MR images, demonstrating normal (left) and abnormal (right-cognitive dysfunction patient) interthalamic adhesion thicknesses in dogs. (Hasegawa et al., 2005. Reproduced with permission from Wiley.)280 As with human AD, there is no known cure for CDS. There are multiple proposed therapeutic and preventative approaches to CDS, with variable evidence of efficacy in improving cognitive function and/or delaying the progression of cognitive decline. These treatments include a variety of drugs (Table 7.4) and dietary supplements/modifications (Table 7.5). There is evidence of efficacy for some of these treatments in dogs. Information pertaining to treatment efficacy for such interventions in cats is mainly anecdotal. The use of oral L-deprenyl (selegiline), an irreversible inhibitor of monoamine oxidase B (MAOB), has been purported to improve cognitive function and slow the progression of the disease in the majority of dogs and cats with CDS. There is considerable variability in the degree of response achieved among patients, however. L-deprenyl is thought to exert its beneficial effects in the brain by restoring dopaminergic balance, as well as enhancing catecholamine levels, and decreasing levels of damaging free radical species. The dosage for dogs is 0.5–1.0 mg/kg every 24 hrs. Cats are administered 0.5 mg/kg every 24 hrs. Most patients will exhibit a positive response within the first month of therapy. Despite apparent positive responses of both canine and feline CDS patients to selegiline, there is some evidence that this drug does not have a significant effect on cognitive function in these patients, or in people with AD. The clinical efficacy studies supporting selegiline use in CDS are based primarily on owner response to questionnaires, rather than on standardized comparative cognitive testing procedures of treated and untreated patients. Since selegiline may produce nonspecific low-level hyperactivity by increasing brain catecholamine levels, the “response” observed by owners may not truly be representative of improved cognitive ability. Selegiline is not considered an effective drug for human AD, due to variable responses and overall minimum improvement of cognitive function. The acetylcholinesterase inhibitor phenserine has exhibited efficacy in improving cognitive function in both dogs with CDS and humans with AD in clinical trials; to the author’s knowledge, this drug is not yet commercially available for dogs. Nicergoline and propentofylline are drugs that theoretically can improve cognitive function by improving cerebral blood flow. There is little to no evidence of efficacy for these two drugs. The noradrenergic-enhancing drugs adrafanil and modafinil have shown some efficacy in improving locomotion and learning in dogs, but seem to further impair memory. Behavioral changes (especially anxiety) in CDS patients may be alleviated with the use of GABA-ergic drugs, such as gabapentin or pregabalin. Other drugs that may alleviate anxiety include buspirone, fluoxetine, and benzodiazepine derivatives. Because inflammatory changes have been identified in the brains of CDS dogs, the use of anti-inflammatory drugs (e.g. carprofen) has also been proposed. A large number of complementary therapies have been suggested for the treatment of CDS, with the primary goals of calming the patient, reducing anxiety, and normalizing the sleep/wake cycle. These include melatonin, valerian root, dog-appeasing pheromone (DAP), and lavender essential oils. The evidence for efficacy of all these complementary therapies is largely anecdotal. Table 7.4 Doses for drugs for behavior therapy of senior pets (oral dosing). a Use single dosing prior to sleep or anxiety-evoking event, up to maximum daily dosing for control of ongoing anxiety. Table 7.5 Ingredients and doses of natural therapeutics for senior pets. There is convincing evidence that providing a diet fortified with antioxidants, mitochondrial cofactors, and essential fatty acids improves cognitive function and delays cognitive decline in dogs with CDS. A commercially available canine diet (Hills b/d) contains a mixture of fruits and vegetables, in addition to vitamins C and E, and mitochondrial cofactors (L-carnitine, DL-α-lipoic acid). Another commercially available diet for dogs (Purina One Vibrant Maturity 7+ Formula) has been shown to improve cognitive function in dogs; this diet contains medium-chain triglycerides (MCTs) which are converted to ketones by the liver. Since reduced brain glucose utilization occurs in CDS and AD, MCTs provide an alternative brain energy source. In addition, MCTs have been shown to increase brain mitochondrial function, increase polyunsaturated fats in the brain, and decrease the level of brain amyloid precursor protein (APP). Although similar commercially available diets for CDS are not available for cats, a number of dietary supplements with antioxidant activity are available for this species. Phosphatidylserine and S-Adenosyl-L-methionine (SAMe) are two natural dietary supplements that have shown some efficacy in the treatment of CDS in dogs. Both of these supplements are thought to have beneficial antioxidant properties. A calcium-buffering protein called apoaequorin has recently been shown to have some efficacy in enhancing learning and attention in older dogs. Though not yet evaluated in dogs and cats, there are several naturally occurring phytochemicals that exhibit anti-amyloidogenic, antioxidative and anti-inflammatory properties. These include resveratrol (found in grapes, red wine, and berries), curcumin (a spice used in several Indian foods), and catechin (found in green tea). Environmental enrichment, such as regular exercise and introduction of new toys, has also been demonstrated to improve cognitive function and delay cognitive decline in dogs with CDS. Progression of CDS appears to be more rapid in castrated versus intact male dogs, suggesting a potential role for hormone replacement therapy in this disease. The prognosis for CDS is guarded. Most affected patients are euthanized within 18–24 mos of the onset of clinical signs, either due to progressive cognitive impairment or unassociated medical problems. The anatomy of the ventricular system of the dog is summarized in Fig. 7.8. The pathophysiology of CNS damage associated with hydrocephalus is complex, and involves the destruction of the ependymal lining of the ventricles, neuronal injury in the cerebral cortex, compromise of cerebral vasculature, and damage to periventricular white matter. The phenomenon of excessive CSF in the ventricular system of the brain occurs commonly in young dogs, especially of the toy and brachycephalic breeds, and less commonly in cats. Congenital hydrocephalus is most commonly reported in dogs, especially small breeds (e.g. Chihuahua, Yorkshire Terrier, Maltese, Boston Terrier, English Bulldog, Toy/Miniature Poodle, Lhasa Apso, Pomeranian, Pekingese). Congenital hydrocephalus may be an autosomal recessively inherited trait in the Siamese cat. The list of potential causes for congenital hydrocephalus (i.e. evident from birth) is diverse and extensive, and involves disturbances to the developing fetus or the neonate, including the following: intraventricular hemorrhage (e.g. dystocia-related); viral infections (e.g. parainfluenza virus in dogs, coronavirus in cats); teratogen exposure; nutritional deficiencies (e.g. vitamin A); and heritable malformations. Traditional/historical theories to explain the ventriculomegaly of congenital hydrocephalus are based on the “bulk flow” concept of CSF accumulation and contend that excessive fluid accumulation results from obstruction of CSF flow within the ventricular system (e.g. mesencephalic aqueduct stenosis) and/or insufficient absorption of CSF into the venous system at the arachnoid villi level. These mechanisms may contribute to some cases of congenital hydrocephalus, but likely are not responsible for the development of this disease in the majority of cases. A more recently proposed theory, called the “hydrodynamic theory,” maintains that the hydrocephalus develops due to abnormal (reduced) intracranial compliance and the resultant effect of this compliance defect on brain capillaries. In the normal animal, individual brain capillaries remain open during the entire cardiac cycle (systole and diastole). This is important because much of the CSF absorption actually occurs at the capillary level, rather than the arachnoid villi. Hydrocephalic patients are believed to have poor intracranial compliance as an underlying disorder that leads to hydrocephalus. The increased capillary pulse pressure caused by decreased compliance leads to a pulsatile transmantle pressure gradient directed from the cerebral tissue toward the lateral ventricles. These abnormal capillary pulsations occur within normal mean intracranial pressure (ICP) limits. The rebound pressure from the recurring gradient as well as hyperdynamic CSF flow in the mesencephalic aqueduct leads to progressive ventricular enlargement. The result is that congenital hydrocephalus often develops within the confines of normal ICP (i.e. normal pressure hydrocephalus). Hydrocephalus, especially if progressive, can cause neurologic dysfunction from compression and stretching of brain parenchyma, as well as from brain ischemia and interstitial edema. Figure 7.8 Schematic illustration depicting the normal ventricular anatomy of the canine brain. Illustration by Michael Simmons. (Dewey and Marino, 2012. Reproduced with permission from Elsevier.)142 It is the rule, rather than the exception, that a specific cause for congenital hydrocephalus is not apparent at the time of clinical presentation; this lack of an active causative process (e.g. inflammation, hemorrhage) helps define this form of hydrocephalus. Hydrocephalus, especially if progressive, can cause neurologic dysfunction from the compression and stretching of brain parenchyma, as well as from brain ischemia and interstitial edema. Many animals, especially of the predisposed breeds, have hydrocephalus based upon ventricular enlargement yet have no discernible neurologic dysfunction. There is generally an inconsistent relationship between the extent of ventricular dilation and clinical signs of disease in most of these breeds, so that clinical hydrocephalus should not be diagnosed on imaging findings alone. One study, however, evaluated both ventricular-to-brain (VB) ratio and basilar artery resistive index (RI) via Doppler ultrasonography in dogs with ventriculomegaly and varying signs of neurologic dysfunction. It was found that RI and VB ratio were both significantly higher in clinically hydrocephalic dogs compared with dogs having ventriculomegaly and no signs of neurologic dysfunction. Combining these measurements provided a sensitivity and specificity of identifying clinical hydrocephalic patients of 77% and 94%, respectively. In addition, it was found that RI changed with changes in neurologic status (but not VB ratio) and that nonclinical dogs with ventriculomegaly and a VB ratio of more than 60% eventually developed clinical hydrocephalus. Although hydrocephalus typically denotes dilation of the internal ventricular system, external hydrocephalus occasionally occurs in people and has been described in dogs and cats. In this text, a patient is considered to have congenital hydrocephalus only if all three of these criteria are met: (1) ventriculomegaly is demonstrated; (2) there is no active, potentially causal disease process identifiable; and (3) the patient exhibits clinical signs of brain dysfunction. Hydrocephalus may exist concurrently with other anomalous conditions that affect the CSF pathways, such as Dandy–Walker syndrome (DWS), Chiari-like malformation (CLM; also termed caudal occipital malformation syndrome, or COMS), and SM (typically associated with CLM). Figure 7.9 Chihuahua with congenital hydrocephalus, exhibiting enlarged calvarium and bilateral ventrolateral strabismus. Diagnosis of congenital hydrocephalus is based upon a combination of characteristic clinical features, demonstration of ventriculomegaly, and the absence of other causes of encephalopathy. Ultrasonography (through open fontanelles or calvarial defects; Fig. 7.10) and advanced imaging (CT/MRI; Fig. 7.11) have largely supplanted more invasive methods of documenting ventriculomegaly (e.g. contrast ventriculography). Electroencephalography (EEG) has been used historically to assist in the diagnosis of congenital hydrocephalus, with affected patients typically exhibiting slow-frequency, high-voltage activity. However, these EEG findings are relatively nonspecific and seldom contribute much to the diagnosis of congenital hydrocephalus. Figure 7.10 Dilated lateral ventricles of a hydrocephalic dog, demonstrated via ultrasonography through an open fontanelle. Figure 7.11 Transaxial T2-weighted MR images of (A) a dog with congenital internal hydrocephalus (Coates et al., 2006; reprinted with permission)96 and (B) a cat with external congenital hydrocephalus. (Dewey et al., 2003. Reprinted with permission from JAAHA, November/December 2003. Copyright © 2003 American Animal Hospital Association (aaha.org). All Rights Reserved.)139 The medical treatment of congenital hydrocephalus (Table 7.6) is aimed at the reduction of CSF production. Oral prednisone, at an initial dosage of 0.25–0.50 mg/kg q 12 hrs, may decrease CSF production. Prednisone should be reduced over several weeks to the lowest possible dosage required to control clinical signs. Furosemide, a loop diuretic, decreases CSF production via inhibition of the sodium/potassium co-transport system. The recommended dose range is 0.5–4.0 mg/kg body weight PO, q 12–24 hrs. The diuretic acetazolamide is a carbonic anhydrase inhibitor and is typically dosed at 10 mg/kg body weight PO, q 6–8 hrs. Omeprazole, a proton pump Table 7.6 Drugs for the medical treatment of congenital hydrocephalus. PO = oral. inhibitor, has been shown to decrease CSF production in dogs by 26%. The oral dose for dogs is 10 mg (dogs weighing less than 20 kg) q 24 hrs and 20 mg (dogs weighing more than 20 kg) q 24 hrs. For all of these drugs, it is recommended that the dose be tapered to the lowest dose needed to control the clinical signs of disease, in order to avoid serious side effects. Anticonvulsant drugs are administered if the patient is experiencing seizure activity. Medical therapy of congenital hydrocephalus may provide some level of disease palliation in mild cases, but often fails in the long term. The potential side effects of long-term corticosteroid and/or diuretic therapy should be considered along with the questionable efficacy of medical therapy for congenital hydrocephalus, when making treatment decisions. Electrolyte depletion (especially potassium) and dehydration are concerns when using diuretics for prolonged time periods, particularly when combined with corticosteroids. The goal of the surgical treatment of hydrocephalus is to continually divert excessive CSF from the ventricles of the brain to either the peritoneal cavity (most commonly performed; Fig. 7.12) or the right atrium of the heart. Both ventriculoatrial and ventriculoperitoneal shunts (Fig. 7.13) have been successfully placed in dogs with congenital hydrocephalus. Ventriculoperitoneal shunt placement is technically more feasible than ventriculoatrial shunt placement, especially in very small patients (Fig. 7.14).The prognosis for dogs and cats with congenital hydrocephalus is variable, but is generally guarded. Medical therapy may be effective in some patients, whereas others require surgical shunting procedures for the long-term control of clinical signs. The prognosis for sustained clinical improvement in neurologic status after surgical shunting procedures varies in the literature from 50 to 90% for dogs. In the author’s experience, the success rate is approximately 75–80%. Potential postoperative surgical shunt complications in dogs and cats include shunt obstruction, shunt dislodgement, mechanical damage to the shunt, and shunt infection. Figure 7.12 Intraoperative image of shunt placement in the lateral ventricle for congenital hydrocephalus. (Coates et al., 2006. Reprinted with permission.)96 Figure 7.13 Three-dimensional CT reconstruction image of a dog with a ventriculoperitoneal shunt placed. Figure 7.14 Surgical positioning and location of incisions for ventriculoperitoneal shunt (VPS) placement (A). Insertion and anchoring (B1, B2) of the rostral end of the VPS. Grid approach (C) to the peritoneal cavity following subcutaneous tunneling of the VPS. (Dewey, 2013. Reproduced with permission from Elsevier.)133 Since the occipital region of the skull and the first two cervical vertebrae develop together embryologically, it makes inherent sense that multiple developmental disorders, as well as combinations of these disorders, should occur in this anatomical region in small animals, as they do in humans. Craniocervical junction abnormalities (CJAs) is an umbrella term that includes Chiari-like malformation (CLM), atlanto-occipital overlapping (AOO), dorsal compression at C1/C2, and atlantoaxial instability. Atlantoaxial instability and dorsal compression at C1/C2 are discussed in Chapter 13. Syringomyelia (SM) refers to the development of fluid cavities within the parenchyma of the spinal cord and can develop as a secondary consequence from any CJA. CLM is the canine analog of Chiari type I malformation of people and has also been termed caudal occipital malformation syndrome (COMS) and occipital hypoplasia. CLM appears to be a very common neurologic disorder in dogs. This disease is almost exclusive to small-breed dogs, with the Cavalier King Charles Spaniel (CKCS) being the most overrepresented. Other CJAs tend to occur in small-breed dogs as well. Occipitoatlantoaxial malformations (OAAMs) are infrequently encountered in dogs and will be only briefly discussed. These disorders have been reported in small- and large-breed dogs and typically involve a shift of the craniocervical junction by one segment. The atlas fuses to the occipital bone and the atlantoaxial joint resembles the atlanto-occipital junction (Fig. 7.15); OAAMs may be asymmetric. Cranial cervical spinal cord and/or brain-stem compression may develop due to direct impingement upon parenchyma from malformed bone and/or instability (e.g. atlantoaxial instability). Because CLM is so prevalent in the CKCS breed, much of the available literature regarding pathophysiology, diagnosis, and treatment is centered on this breed. CLM has generally been considered a congenital malformation of the caudal occipital region of the skull, leading to overcrowding of the caudal fossa and compression of the cervicomedullary junction at the level of the foramen magnum (Fig. 7.16). However, the anatomical abnormalities associated with CLM are far more complicated than simply a malformed skull in the caudal-most aspect of the occipital bone region causing a physical constriction near the foramen magnum. There is convincing evidence in CKCS dogs that there is a mismatch between the volume available in the caudal fossa region (CF, also referred to as the caudal cranial fossa) and the parenchyma (cerebellum and brain stem) that resides within this volume; in other words, there is too much brain parenchyma in too small a space in the CF of CKCS dogs (compared to other small-breed dogs and Labrador Retrievers). This mismatch between parenchyma and available volume has also been demonstrated in the cranial fossa (rostral and middle fossae) of CKCS dogs. Increased ventricular size, increased relative parenchymal volume in the CF (as a percentage of total brain parenchymal volume), and increased relative cerebellar volume have all been associated with increased likelihood of the presence of SM in the CKCS breed. Increased syrinx width has also been associated with increased ventricular size and relative CF parenchymal volume in this breed. There is some evidence in the CKCS breed that the CF volume itself is often too small compared with other dog breeds. Other anatomic abnormalities of the skull reported in dogs with SM include minute or absent frontal sinuses and abnormally small jugular foramen volumes. For this latter abnormality, it is hypothesized that the constricted venous drainage from the brain due to small jugular foramina leads to intracranial venous hypertension and increased ICP; this would lead to an increased pressure differential between cranial and spinal compartments and an increased likelihood of SM development. In AOO cases, the atlas (C1) is cranially displaced into the foramen magnum, and there is overlap of the occipital bone and the atlas (Fig. 7.17). This displacement tends to compress the caudal aspect of the cerebellum and elevate and compress the caudal medulla (medullary kinking). AOO is likely a form of basilar invagination. Basilar invagination is a human craniocervical junction disorder in which the atlas and/or axis (C2) telescope toward the foramen magnum. It is possible that some cases of atlantoaxial instability in dogs are also analogues of basilar invagination. Since AOO has only recently been described, the bulk of the literature on the topic of CJAs refers to CLM. There is convincing evidence in the CKCS breed that CLM is a heritable disease, although the exact mode of inheritance has not been determined. Other breeds affected by CLM or similar disorders (like AOO) include Brussels Griffon (Griffon Bruxellois), Miniature/Toy Poodle, Yorkshire Terrier, Maltese, Pug dog, Pomeranian, Chihuahua, Staffordshire Terrier, Shih Tzu, Miniature Dachshund, Miniature Pinscher, French Bulldog, Boston Terrier, and Pekingese. Anecdotal reports describe a disorder similar to CLM in several brachycephalic cats. Based on combined MR and CT imaging of dogs with CJAs, there is evidence that a substantial proportion (nearly 30%) of dogs diagnosed with CLM on MR images may actually have AOO as the main anatomic abnormality causing compression at the cervicomedullary junction. Bone is poorly visualized on MR images, but CT images clearly delineate what bony structures are causing compression at the cervicomedullary junction. In short, it is likely that many dogs with constrictive disorders at the cervicomedullary junction diagnosed via MR imaging as having CLM may actually have AOO. The vast majority of dogs with CLM or AOO have SM (usually noted in the cervical spinal cord, but often in multiple regions of the spine if these regions are also imaged), an accumulation of fluid within the spinal cord, as a consequence of the malformation. In patients with CLM or AOO, there tends to be some level of cerebellar compression as well as constriction of the cervicomedullary junction in the vicinity of the foramen magnum. With chronic bony compression at the cervicomedullary junction and probable turbulent CSF flow and pressure changes in this region, it is thought that the underlying meninges become hypertrophied with time. In both humans with Chiari type I and dogs with CLM, there is pathological evidence of dural fibrosis in the region of the malformation. In CLM, as in Chiari type I of people, the caudal aspect of the cerebellum is often projecting into or through (herniation) the foramen magnum, contributing to the obstruction of CSF flow between intracranial and spinal compartments. Progressive alterations in pressure dynamics between the intracranial and spinal compartments are believed to be responsible for the development of clinical signs of CLM. Although aberrant pressure dynamics due to the obstruction of CSF pathways at the level of the foramen magnum are generally agreed to be associated with SM in CJAs, the exact mechanism of this development is unknown and there are multiple theories proposed to explain it. Many of these theories operate on the probably incorrect premise that the SM fluid is CSF which is forced into the central canal of the spinal cord. The newer theories suggest that the SM fluid is actually derived from extracellular fluid from the cord itself, either driven into the central canal via a pulse pressure wave from behind the foramen magnum obstruction and/or drawn into the central canal via a centrifugally directed hydrostatic pressure force within the spinal cord. In normal dogs, there is pulsatile CSF flow across the foramen magnum from intracranial subarachnoid space to cervical spinal subarachnoid space and back again during systole and diastole, respectively. With an obstruction at the foramen magnum and/or substantial pressure differential (due to overcrowding of the caudal fossa), as occurs with CLM, CSF does not flow well in either direction. In this scenario, the pressure exerted during systole may drive either CSF or a pressure wave from the intracranial compartment into the central canal region of the cranial cervical spinal cord, causing it to progressively expand. This has been referred to as the “water-hammer effect.” Another theory proposed is that CSF is “sucked” into the central canal region of the cervical spinal cord, especially during maneuvers that lead to sudden increases in intrathoracic an intra-abdominal pressure (e.g. coughing, sneezing, exercising). These Valsalva maneuvers lead secondarily to increased intracranial and intraspinal pressure via epidural venous distension. Because ICP is higher than in the cervical cord region, CSF fluid is drawn into the cervical cord when there are rapid increases in pressure. Pressure within the spinal compartment tends to increase more rapidly in the lumbar versus cervical regions, further promoting CSF movement into the cervical cord via this “suck” effect. The “slosh” phenomenon may also be involved in expansion of a syrinx. With distension of epidural veins during Valsalva events, CSF flows more freely within the syrinx than in the compressed subarachnoid space. Therefore, sudden CSF pressure waves cause CSF within the syrinx cavity to “slosh” around, fissuring surrounding parenchyma and enlarging the syrinx. The combination of spinal epidural vein distension (and the resultant pressurization of the subarachnoid space) and obstruction to CSF flow from the cervical spine to the intracranial compartment may also result in forcing subarachnoid CSF down perivascular spaces into the spinal cord parenchyma, progressively enlarging the syrinx. It has also been proposed that the displaced caudal cerebellum acts like a “piston.” The piston theory proposes that the displaced cerebellum moves further caudally during systole, obstructing the subarachnoid space at the foramen magnum and exaggerating the systolic pulse pressure wave that is transmitted from intracranial to spinal compartments; this further forces CSF through perivascular spaces into the syrinx. Although all of the above theories may contribute to the development of a syrinx, none of them is an adequate explanation for this phenomenon. It has been shown that the syrinx usually has a higher pressure than the subarachnoid space, which would argue against theories that propose CSF is being forced or sucked into a low-pressure system from a higher-pressure system. In addition, syrinx fluid is not identical to CSF fluid: it has a lower protein concentration and is more consistent with extracellular fluid. Several related theories have been proposed that are more likely to adequately explain the pathogenesis of SM formation with CLM or Chiari type I malformation. The “intramedullary pulse pressure” theory proposes that the spinal cord parenchyma distal to the foramen magnum compression (or high-pressure region) is subjected to distending forces that tend to pull the tissue in an outward or centrifugal direction. The combination of transmittal of the systolic pulse pressure wave to the spinal cord parenchyma (due to obstruction of the subarachnoid space) and decreased subarachnoid space pressure in the spinal cord region (due to obstruction of the subarachnoid space rostral to the foramen magnum) leads to this mechanical distension. Over time, the distension leads to a cavity formation (syrinx), which is filled with extracellular fluid. The “Venturi effect” describes a similar mechanical spinal cord distension caused by increased CSF velocity distal to an obstruction. The obstruction (i.e. foramen magnum occlusion) causes a narrowing of the subarachnoid space and a resultant increased fluid velocity distal to the obstruction. This increased velocity lowers the hydrostatic pressure, producing a centrifugally directed suction effect, leading to spinal cord distension. This theory also assumes that the accumulated fluid in the syrinx is extracellular fluid and at a higher pressure than the subarachnoid space. Finally, there is a “vascular” theory to explain the development of SM in CLM cases. With increased CSF pressure in the intracranial compartment vs. the spinal compartment due to foramen magnum obstruction and/or caudal fossa overcrowding (especially during Valsalva maneuvers and systole), the venous and capillary beds become collapsed in the intracranial region and distended in the cervical spinal cord region (caudal to the obstruction). This occurs because CSF and venous pressure normally remain closely matched in both cranial and spinal compartments, and the venous system does not become obstructed as does the subarachnoid space with foramen magnum obstruction. With foramen magnum compression, the transmural pressure (difference between intravascular and interstitial pressure) of the venous and capillary system on either side of the obstruction is no longer uniform throughout the spinal cord. The uneven vascular expansion and contraction that ensues causes damage to the surrounding spinal cord. This hydrostatic stress-mediated damage to the spinal cord disrupts the blood-spinal cord barrier, promoting the accumulation of extracellular fluid within the spinal cord (i.e. syrinx development). Common to all theories of syrinx development in CLM is the causative factor of the obstruction or impeding of normal CSF flow at the foramen magnum. Figure 7.15 Three-dimensional CT reconstruction image of a dog with an occipitoatlantoaxial malformation before (A) and after (B) surgical stabilization. Figure 7.16 Schematic illustration of normal canine caudal fossa anatomy (A) and abnormal caudal fossa anatomy (B) associated with Chiari-like malformation. (Dewey, 2013. Reproduced with permission from Elsevier.)132 Figure 7.17 Three-dimensional reconstructed CT image (A) and midsagittal T2-weighted MR image (B) of a dog with atlanto-occipital overlap (AOO). (Dr. Dominic Marino, Long Island Veterinary Specialists, Plainview, NY, 2014. Reproduced with permission from Dr. Dominic Marino.) Most dogs with CLM and other CJAs (AOO, AA instability) are presented for evaluation as young adults. Most of the OAAMs reported in dogs were presented within the first year of life. The typical age range at presentation for CLM appears to have changed over time, with many dogs developing clinical signs within the first year of life. In general, though the age range at clinical presentation is broad, most dogs present by the time they are 4 yrs old. Dogs that are presented at less than 2 yrs of age often have more severe clinical signs than older dogs. In recent years, there have been an increasing number of younger (< 1 yr of age) patients presenting with CLM or related disorders; whether this trend reflects an increasing severity of the disorder with subsequent generations, increased awareness of the veterinary community and hence earlier diagnosis, or a combination of these two factors is unknown. In the author’s experience, AOO is more likely than CLM in toy- and small-breed dogs (e.g. Yorkshire Terrier, Chihuahua), whereas CLM is more likely in the CKCS breed. Similar to Chiari type I of humans, there is a wide spectrum of possible neurologic presentations for dogs with CLM, including cervical myelopathy, cerebellovestibular dysfunction, and forebrain dysfunction (e.g. seizure activity). By far, evidence of cervical dysfunction and cerebellovestibular dysfunction are the most common and are often both present (e.g. multifocal CNS disease). Most of the CLM cases that the author encounters are presented for signs referable to the cervical region (e.g. neck pain, scratching activity) and subtle signs of central vestibular dysfunction are apparent on neurologic examination (i.e. not noticed by the owner). Occasionally, dogs with CLM and cervical SM present with a specific variant of cervical myelopathy called central cord syndrome. In this syndrome, the outwardly expanding syrinx causes more lower motor neuron (LMN) damage to the thoracic limb musculature than white-matter damage (to pelvic limbs); the result is thoracic limb paresis (often LMN in nature) that is notably worse than pelvic limb paresis. In some cases, the pelvic limbs may appear normal. Some specific clinical findings in dogs with CLM include cervical and cranial hyperesthesia, decreased menace responses with normal vision, positional ventrolateral strabismus, thoracic limb weakness, pelvic limb ataxia, persistent scratching (at the head, neck, and shoulder region, often without making skin contact), scoliosis, facial nerve paresis/paralysis (unilateral or bilateral), and hearing abnormalities. The persistent scratching activity and scoliosis are fairly unique clinical signs associated with SM. In the author’s experience, these are more commonly encountered in the CKCS breed than in other breeds with CLM and SM. The scratching activity is believed to be due to the syrinx interfering with spinothalamic tracts and/or dorsal horn neurons, resulting in abnormal sensations (dysesthesia/paresthesia). Scoliosis (torticollis) is most likely due to asymmetric syrinx damage to sensory proprioceptive neurons innervating cervical musculature; an alternative, less likely hypothesis is syrinx damage to LMNs innervating cervical musculature. Scratching activity and neck discomfort often are exacerbated by abrupt weather changes, stress or excitement, and physical contact with the neck/shoulder region (e.g. collar). The presence of both pain and scoliosis has been shown to be significantly correlated with syrinx width in CKCS dogs with SM secondary to CLM. It is important to realize that, especially in the CKCS breed, other conditions may account for some of the clinical signs. There are several reports documenting a sizeable proportion of CKCS dogs with either CLM or CLM and SM that were regarded as clinically normal. Since these dogs were recruited for various imaging studies, they are not randomized samples of the CKCS population. Nonetheless, the percentage of clinically normal CKCS dogs with CLM and MR evidence of SM has been reported to range between approximately 25 and 70%; in one study, it was found that the low end of this range was associated with younger dogs (1 yr or less) and the higher end with older dogs (3 yrs or more). As mentioned, syrinx width tends to be smaller in asymptomatic dogs vs. symptomatic dogs. In addition, the syrinxes of asymptomatic dogs tend to be more symmetric and are less likely to extend into the dorsal horn region of the spinal cord. An enigmatic ear problem of the CKCS breed, called primary secretory otitis media (PSOM), has been described. Clinical signs of PSOM include apparent pain around the head and neck area, scratching of the head and neck, facial paralysis, and head tilt. Idiopathic epilepsy is also a prevalent disorder in the CKCS breed. Seizures have been reported to occur in 10 to 12% of humans with Chiari type I malformation; in the author’s experience, seizure activity is an infrequent concurrent occurrence in CLM cases, and it is usually not possible to distinguish whether this is due to CLM or concurrent idiopathic epilepsy. Congenital deafness is also well described in the CKCS breed. The severity and rate of progression of CLM in dogs is variable, ranging from asymptomatic (i.e. finding evidence of CLM while imaging for some other reason) to extreme pain and debilitation with rapid worsening. In addition, some dogs with CLM have other concurrent disorders (e.g. disc extrusion, inflammatory brain disease) that could explain observed clinical signs. In such situations, it may be difficult to discern if the CLM is the main problem, contributory, or an incidental finding. Diagnosis of CLM and other CJAs is made by MR imaging (Fig. 7.18). MRI is also the preferred imaging modality for diagnosing SM (Fig. 7.19). The malformation is best visualized on a midsagittal view (preferably T2-W), which includes the caudal fossa and cranial cervical cord. Consistent findings on MR imaging indicative of CLM are attenuation/obliteration of the dorsal subarachnoid space at the cervicomedullary junction and rostral displacement of the caudal cerebellum by the occipital bone. Other common MRI findings in CLM include SM (usually C2 level caudally), herniation of the caudal cerebellum through the foramen magnum (Fig. 7.20), and a “kinked” appearance of the caudal medulla. In one study of CKCS dogs, it was found that a flexed head position during MR imaging was more likely to exacerbate the degree of cerebellar herniation than an extended head position. Phase-contrast MRI (cine-MRI) is often used to measure CSF flow in humans with Chiari type I malformation, and has also been evaluated for use in dogs with CLM. In dogs, decreased flow at the foramen magnum and C2/C3 vertebral level, as well as turbulent flow and jets, were predictive of the presence of SM. Dogs with MRI findings consistent with CLM will occasionally have evidence of other congenital disorders such as intracranial arachnoid (quadrigeminal) cyst, malformation of the C1 and/or C2 vertebrae, and hydrocephalus. In the author’s opinion, most small-breed dogs normally have large lateral ventricles as a breed characteristic (ventriculomegaly) and are not hydrocephalic. As mentioned previously, the specific anatomic structure causing a constrictive effect at the craniocervical junction can be difficult to ascertain on an MR image. The author and colleagues routinely perform CT scans through the abnormal craniocervical junction following MR imaging to determine whether the abnormality is truly indicative of CLM or something else (e.g. AOO). Several potential methods of screening for the presence or absence of CLM/SM have been evaluated in dogs, including ultrasound, brain-stem auditory evoked response (BAER) testing, and thermography. None of these methods has emerged yet as a reliable screening tool; in addition, screening for a breed such as the CKCS that has such a high prevalence of the CLM/SM disorder with a high proportion of asymptomatic dogs may be of limited value. In the Brussels Griffon breed, one study demonstrated that a specific ratio based upon measurements from skull radiographs was 87% sensitive and 78% specific for predicting the presence of CLM (as diagnosed on MR images). In the absence of concurrent disease processes, CSF analysis is usually normal in CLM/SM; occasionally, a mild mononuclear pleocytosis will be apparent, however. In one study of CKCS dogs with CLM with or without SM, it was found that total nucleated cell counts (TNCC) and protein concentrations in CSF were significantly higher in dogs with SM versus dogs without SM. In that study, there was also a positive association between TNCC and syrinx size. Figure 7.18 Midsagittal T2-weighted MR image of a normal small-breed dog (A) and a dog with Chiari-like malformation (B). Figure 7.19 Sagittal (A) and transaxial (B) cervical spinal cord MR images demonstrating a large syrinx cavity in a dog with Chiari-like malformation. Figure 7.20 Midsagittal T2-weighted MR image of a dog with Chiari-like malformation, demonstrating cerebellar herniation through the foramen magnum. Treatment of CLM and other CJAs can be divided into medical and surgical therapy. In people with symptomatic Chiari type I malformation, surgical therapy is considered the treatment of choice, with foramen magnum decompression (FMD) being the preferred surgical procedure. Adjunctive surgical procedures are occasionally performed in people who have had a suboptimal response to FMD; such procedures usually involve placement of a shunt to divert SM fluid from the spinal cord region to another location for absorption (e.g. pleural or peritoneal cavity, subarachnoid space). Although there is a high degree of success in the surgical management of Chiari type I malformation in people, there is a re-operative rate varying from 8 to 30% for FMD; the most common problem necessitating re-operation is excessive scar tissue formation at the FMD site causing compression at the cervicomedullary junction, effectively recreating the original disease state. Medical therapy for dogs with CLM generally falls into three categories: analgesic drugs (implies relief of dysesthesia/paresthesia also), drugs that decrease CSF production, and corticosteroid therapy. By far the most useful drug available for the relief of scratching activity associated with SM has been gabapentin (10 mg/kg body weight PO, q 8 hrs). It has been shown that neuropathic pain is accentuated over time due to up regulation of the α2δ-1 subunit of voltage-gated calcium channels in dorsal root ganglion neurons and dorsal horn nociceptive neurons of the spinal cord. Gabapentin, and the newer gabapentin analog, pregabalin, are believed to exert their antinociceptive effects by selectively binding to the α2δ-1 subunit and inhibiting calcium influx in these neurons. Side effects of gabapentin are minimal, usually restricted to mild sedation, pelvic limb ataxia, and weight gain. Pregabalin is the “next generation” of gabapentin with a higher affinity for the α2δ-1 subunit; the elimination half-life in dogs is about 7 hrs vs. 3–4 hrs for gabapentin. In the author’s experience, oral pregabalin dosed at 2.0 mg/kg q 12 hrs is more effective in treating dogs with CLM/SM than gabapentin. Side effects of pregabalin have been limited to sedation and weight gain. Orally administered opiate drugs are sometimes helpful in alleviating neck and head pain in CLM dogs. The author has had success using oral tramadol (2–4 mg/kg, q 8–12 hrs). A number of drugs aimed at decreasing CSF production have been used in CLM patients, in an effort to diminish the CSF pulse pressure. All information regarding efficacy of these drugs is anecdotal. They include omeprazole (a proton pump inhibitor), acetazolamide (a carbonic anhydrase inhibitor), and furosemide (a loop diuretic). More specific information regarding these drugs is covered in the congenital hydrocephalus discussion. Corticosteroids are often used in the medical management of CLM. Potential benefits include anti-inflammatory effects, decreased CSF production, and decreased substance P (a nociceptive neurotransmitter) expression in spinal cord dorsal horn neurons. An initial anti-inflammatory dose of 0.5 mg/kg PO, q 12 hrs is often effective in controlling clinical signs. This dose should be tapered, if at all possible, to an every-other-day schedule within the first month of therapy. In most cases of CLM, medical therapy will diminish the severity of clinical signs, but resolution is unlikely. The preferred surgical procedure for the treatment of CLM in dogs is FMD. Based on two similar reports, short-term surgical success rates with FMD in dogs with CLM are approximately 80%. One report found an inverse relationship between the length of time clinical signs were present prior to surgical intervention and the extent of postoperative improvement. Unfortunately, there appears to be a disease relapse rate ranging from 25 to 47% of cases; most of these relapses are suspected to be due to excessive postoperative scar tissue formation at the FMD site. In most cases, clinical signs of pain are routinely relieved with surgery, but scratching activity tends to persist. The author and colleagues have adapted a cranioplasty procedure used in human FMD surgery to discourage excessive postoperative scar tissue from recompressing the operative site (Fig. 7.21). Based on over 300 cases, the titanium mesh/PMMA plate procedure has reduced the re-operative rate to less than 1% (unpublished data). Syringosubarachnoid shunting has been evaluated retrospectively in a small cohort of dogs (11 dogs) with CLM/SM; nine dogs were judged as neurologically improved at 6 mos follow-up, and seven dogs were still alive a mean of 2.6 yrs post surgery. Figure 7.21 Schematic illustration of foramen magnum decompression/cranioplasty procedure for Chiari-like malformation (A–C), and postoperative three-dimensional CT reconstruction (D) demonstrating the plate in place. (Dewey, 2013. Reproduced with permission from Elsevier.)132 There is sparse information regarding the prognosis for CLM in dogs with both surgical and nonsurgical management. Most dogs with CLM will respond favorably to medical therapy, although in many cases this response is temporary. In one group of 10 CLM dogs treated medically, five dogs were euthanized within 2 yrs due to disease progression and diminished responsiveness to therapy. In another study, 36% of CLM dogs treated medically were euthanized due to clinical signs of their disease at mean of 1.7 yrs from the time of diagnosis. In one longer-term study evaluating the response to nonsurgical management, 48 CKCS dogs with CLM (+/–SM) were prospectively followed for a mean of 39 mos; despite the fact that the majority (75%) of these dogs continued to deteriorate clinically over time (25% remained static or improved), 75% of the total (36 dogs) were still alive at the end of the study period. Although the surgical success rate is generally favorable for CLM in dogs, many dogs still require some degree of medical management and some dogs do not improve—even in the absence of postoperative scar tissue compression of the FMD site. It is hoped that future modifications of surgical procedures (e.g. larger decompressions to account for intracranial cavity volume/parenchyma mismatch) or combinations of procedures (e.g. FMD with syrinx shunting) will ameliorate this problem. In general, the overall prognosis for CLM and related disorders in dogs is guarded to good for sustained improvement in clinical signs. Intracranial arachnoid cyst (also termed intra-arachnoid cyst and quadrigeminal cyst) is a developmental brain disorder in which CSF is thought to accumulate within a split of the arachnoid membrane during embryogenesis. The developing neural tube is surrounded by a loose layer of mesenchymal tissue called the perimedullary mesh; this tissue eventually becomes the pia and arachnoid layers of the meninges. In normal development, pulsatile CSF flow from the choroid plexuses is thought to divide the perimedullary mesh into pia and arachnoid layers, effectively creating the subarachnoid space. It is postulated that some aberration of CSF flow from the choroid plexuses during this stage of development forces a separation within the forming arachnoid layer, eventually leading to the creation of an IAC. The intra-arachnoid location of IACs has been demonstrated via light and electron microscopy in people. The mechanisms by which an IAC continues to expand with fluid are unknown, but several theories have been proposed. Fluid may be secreted by the arachnoid cells lining the cyst cavity. There is evidence that cells lining the IAC may have secretory capacity. There may also be fluid movement into the cyst via an osmotic pressure gradient. Considering that the fluid within the IAC is nearly identical to CSF, this theory is unlikely. In addition, there have been documented cases in people in which small slits exist between the IAC and the subarachnoid space; these slits act as one-way valves, diverting CSF into the cyst during systole which cannot return to the subarachnoid space during diastole. Although IAC has been reported to occur in several locations in humans, all reported canine cases have been in the caudal fossa. Because IAC is typically associated with the quadrigeminal cistern in dogs, these accumulations of fluid are often called quadrigeminal cysts in this species. A similar structure was reported in a 1-yr-old cat, based upon MR imaging of the patient. The author has also observed a similar cystic condition in a kitten. Also termed intracranial intra-arachnoid cyst, IAC accounts for 1% of all intracranial masses in people, and has been sporadically reported in dogs. IAC is often an incidental finding in humans; it has recently been suggested that this may also be the case for IAC in dogs. In the veterinary literature, there have been nine clinical reports of IAC in dogs, with a total of 53 cases. The IAC was suspected to be an incidental finding in approximately one-third to over one-half of the reported cases. The vast majority of reported IAC cases in dogs have been small breeds, with a predominance of brachycephalic dogs. The breeds reported to date include Shih Tzu (12), Maltese (4), Pug (4), CKCS (4), Yorkshire Terrier (4), Lhasa Apso (4), Chihuahua (3), Staffordshire Bull Terrier (3), Bulldog (3), Pekingese (2), West Highland White Terrier (2), and one each of Bichon Frise, Pomeranian, Cairn Terrier, Jack Russell Terrier, Terrier mix, Beagle, Miniature Schnauzer, and German Shorthaired Pointer. Figure 7.22 T2-weighted midsagittal (A) and dorsal T1-weighted (B) MR images of an intracranial arachnoid cyst. Figure 7.23 Intraoperative view (A) of the exposed wall of an intracranial arachnoid cyst and postoperative ventrodorsal radiograph (B) demonstrating placement of a cystoperitoneal shunt in a dog with an intracranial arachnoid cyst. (Dewey et al., 2007. Reproduced with permission from Wiley.)140 Figure 7.24 T2-weighted MR image (transaxial view) of a lissencephalic dog’s brain. Note the absence of sulci on the cerebral surface. (Dr. G. Kort, 2014. Reproduced with permission from Dr. G. Kortz.) There are a number of rarely reported malformations of the brain, most of which are not compatible with life. Exencephaly is the protrusion of brain tissue through a calvarial defect without a meningeal or skin covering. Meningoencephalocele refers to a similar protrusion that maintains both meningeal and skin coverings. Hydranencephaly describes the absence or near absence of cerebral cortical tissue, and may be difficult to discern from very severe hydrocephalus. Anencephaly is the total or partial absence of brain tissue and calvarium. Porencephaly is a condition characterized by cystic cavities in the cerebrum, which may communicate with the ventricular system. Holoprosencephaly describes a single, nondivided cerebrum. This disorder has been described in a Miniature Schnauzer and has been associated with hypodipsic hypernatremia in this breed. Cyclopian malformation refers to the development of a single, median-positioned eye. Numerous etiologies have been proposed for these disorders, including heritable defects, infectious agents, toxin exposure, and nutritional imbalances. Meningoencephaloceles have been described in Burmese kittens as part of a heritable craniofacial malformation syndrome. Feline parvovirus (panleukopenia virus) has been implicated as a cause of hydranencephaly and porencephaly in kittens. Agenesis of the corpus callosum and cyclopian malformation have been described in kittens exposed to griseofulvin during gestation. Exencephaly may occur when kittens are exposed to griseofulvin, methylmercury, or hydroxyurea during gestation. A number of cystic intracranial developmental lesions have been reported in the veterinary literature. The majority of these reports have been of epidermoid cysts; dermoid cysts, choroid plexus cyst, and epidural mucocele have also been reported. Epidermoid cysts (cholesteatomas) and dermoid cysts have been reported primarily in the cerebellomedullary angle region of dogs of various ages. Most of these dogs had clinical signs of cerebellovestibular dysfunction, though a few were incidental necropsy findings. Epidermoid and dermoid cysts are thought to be due to a failure of complete separation of neuroectoderm from non-neural (epithelial) ectoderm during embryogenesis (i.e. neural tube closure). Epithelial ectoderm with (dermoid cyst) or without (epidermoid cyst) adnexal structures (e.g. hair follicles, sebaceous glands) becomes entrapped within the CNS, and forms a slowly expanding cystic mass. The lumen of an epidermoid cyst contains a mixture of cholesterol, keratinocytes, and keratinaceous debris; dermoid cyst lumens contain combinations of fat, hair, and sweat secretion. Leakage of cyst contents into the surrounding tissue can lead to aseptic meningitis, termed Mollaret’s meningitis in people. Dogs and cats with these miscellaneous brain malformations are usually either stillborn, die, or are euthanized shortly after birth. Epidermoid cysts are usually diagnosed in mature dogs, usually young adults. The reports of dermoid cysts in dogs have also been seen in adults. The CT and MRI appearance of epidermoid cysts has been described in dogs, as has the MRI appearance of dermoid cysts in dogs. These imaging findings are similar to what is reported in people for these disorders. Epidermoid cysts appear to be hypoattenuating on CT images, hypointense on T1-weighted (T1-W) MR images, and hyperintense on T2-W MR images; there is also peripheral or ring-type enhancement of the cyst wall with contrast administration. Sustained hyperintensity of the cyst lumen on FLAIR imaging for epidermoid cysts (due to a lack of free, unbound water protons within the cyst) helps to distinguish them from other cystic-appearing diseases like IACs (Fig. 7.25). Dermoid cysts tend to be hyperintense on T1- and T2-W MR images, due to the fat content within the cyst lumen. The majority of the histopathologic descriptions to date of epidermoid and dermoid cysts have been necropsy reports. Surgical removal of a caudal fossa epidermoid cyst has been described in a dog, but the dog died in the postoperative period. Successful removal of a choroid plexus cyst from the caudal fossa of a dog has also been reported. The successful surgical management of meningoencephalocele has also been described in both the dog and the cat. Figure 7.25 Sagittal T2-weighted (A), transaxial T1-weighted (B), T1-weighted with contrast (C), and FLAIR (D) images of an epidermoid cyst in a dog’s brain. (Dr. Jennifer L. Bouma, DACVR, 2014. Reproduced with permission of Dr. Jennifer L. Bouma.) A cat with intracranial epidural mucocele recovered fully following the surgical removal of the cystic structure. There are no effective treatments for the majority of these disorders and the prognosis is often guarded to poor. A major function of the liver is to filter out potentially toxic substances received from the gastrointestinal tract (via the portal venous system) so that these substances do not gain access to the general circulation. When this function is compromised due to either hepatic failure or portosystemic shunting, or both, clinical signs of encephalopathy may result. The pathogenesis of hepatic encephalopathy is complex. Proposed causative factors include gut-derived toxins—such as ammonia, skatoles, indoles, and short-chain fatty acids—that reach the systemic circulation and cause neurotoxicity; alteration in brain neurotransmitter balance and/or production of “false neurotransmitters” due to increased circulating levels of aromatic amino acids; and circulating benzodiazepine-like substances that act on brain GABA receptors. There are several documented neurotransmitter imbalances associated with hepatic encephalopathy, including decreased glutamatergic tone and increased GABA-ergic tone. Profound changes in brain serotonin metabolism have recently been described in hepatic encephalopathy. These aberrations involve increased serotonin production from tryptophan (probably related to hyperammonemia), increased serotonin turnover (metabolism by monoamine oxidase system), and decreased levels of serotonin transport proteins and neuronal binding sites. The overall effect is thought to be a reduction of serotonergic neurotransmission. Most cases of hepatic encephalopathy are due to congenital portosystemic shunts (PSS), which are aberrant vascular communications between the portal and systemic venous systems (i.e. bypassing the liver). The majority of these shunts are extrahepatic (located outside the liver parenchyma) versus intrahepatic (within the liver parenchyma). Extrahepatic shunts typically occur in small- and toy-breeds of dogs (e.g. Yorkshire Terriers, Miniature Schnauzers) and less commonly in cats, whereas intrahepatic shunts are more common in larger dog breeds (e.g. Labrador Retrievers, Irish Wolfhounds). In one report, the median age for dogs with extrahepatic PSS was 12 mos, and over one-third of the cases were over 2 yrs of age at diagnosis. The Yorkshire Terrier is the most commonly reported breed with extrahepatic PSS. There are a number of breeds that are predisposed to PSS, including Havanese, Yorkshire Terrier, Maltese, Dandie Dinmont Terrier, Pug, and Miniature Schnauzer. There is probably a hereditary basis to PSS, and there is evidence to that effect for Yorkshire Terrier, Cairn Terrier, and Irish Wolfhound breeds. Multiple acquired extrahepatic shunts can form as a result of chronic portal hypertension in dogs and cats with long-standing hepatic disease. A congenital disorder termed hepatic microvascular dysplasia (HMD) has been described in which there are suspected to be multiple microscopic shunting vessels within the liver that bypass the hepatic sinusoidal system, rather than a grossly visible anomalous vessel. This appears to be most common in small and toy breeds of dogs (e.g. Cairn Terriers may be predisposed) and cats. Animals with PSS may also have HMD concurrently. Other causes of hepatic encephalopathy include congenital arteriovenous fistulas, and acquired hepatic disorders (e.g. toxin-induced, infectious, chronic active hepatitis, neoplasia). Clinical signs of hepatic encephalopathy include obtunded mental status, abnormal behavior, compulsive pacing, head-pressing, visual deficits, and seizure activity. Other clinical signs consistent with hepatic failure (e.g. weight loss or failure to gain weight, anorexia, vomiting, diarrhea, PU/PD) are often present. Cats with PSS are more likely to seizure than dogs, and often exhibit ptyalism as a characteristic clinical sign. Patients with PSS usually develop clinical signs associated with hepatic insufficiency within the first year of life, but adult animals are occasionally encountered. Dogs and cats with HMD (without PSS) and acquired hepatic disorders are more likely to develop clinical signs as adults (over 1 yr). Although seizure activity may be a component of the hepatic encephalopathy displayed by PSS dogs, it is infrequent. In one large retrospective study of 168 dogs with single extrahepatic shunts, only one dog had evidence of seizure activity. However, an uncommon (approximately 5% of cases) yet often fatal postoperative complication of PSS attenuation in dogs is seizure activity, which is often severe (i.e. clusters, status epilepticus). Most of these patients do not have seizure histories prior to surgery, typically begin seizuring within the first few days postoperatively, and usually are profoundly encephalopathic. A similar phenomenon has been described in a small number of cats following PSS surgery. Following shunt ligation in some dogs, abrupt changes in brain serotonin levels may also be involved in the development of seizures. Diagnosis of hepatic encephalopathy is based upon documenting hepatic dysfunction in a patient with neurologic deficits typical of a metabolic encephalopathy. Bloodwork abnormalities (microcytic red blood cells, low blood urea nitrogen [BUN], low albumin, etc.) often point to a liver problem. Liver enzymes (e.g. alanine aminotransferase [ALT], serum alkaline phosphatase [SAP]) are typically normal to slightly elevated in PSS and HMD patients but are often elevated in acquired hepatic disorders. Ammonium biurate crystals may be evident on urinalysis in PSS patients. Liver function tests, such as serum bile acid and ammonia levels, are often abnormally elevated. There are multiple methods (e.g. mesenteric portography, abdominal ultrasound) available to demonstrate the existence of PSS, but a more commonly used method is per-rectal scintigraphy. A radioactive compound called technetium (99mTc) is administered rectally, and the patient is placed under a gamma camera to measure radiation activity as the substance is absorbed from the colon. If the radioactivity is first detected in the heart and lungs, rather than the liver, this indicates the presence of PSS (Fig. 7.26). Three-dimensional CT angiography (Fig. 7.27) is also useful in identifying PSS vessels as well as providing useful structural information for surgical planning. Hyperintense lesions in the basal nuclei (particularly the pallidum and putamen—the lentiform nuclei) on T1-W MR images are well described in humans with hepatic encephalopathy. The hyperintense regions are believed to be due to manganese deposition in these regions of the brain. Similar MRI lesions have been described in dogs and cats with PSS (Fig. 7.28), in addition to widened sulci. Figure 7.26 Normal (A) and abnormal (B) per-rectal scintigraphy studies in dogs. Note the lack of radioactivity in the liver of the dog with a PSS (B) compared to the liver of a normal dog (A). (Dr. Anne Bahr, 2014. Reproduced with permission from Dr. Anne Bahr.) Figure 7.27 Three-dimensional CT angiography study of a dog with a PSS. (Dr. P. Scrivani, Cornell University, 2014. Reproduced with permission from Dr. P. Scrivani.) Figure 7.28 Transaxial T1-weighted (A) and T2-weighted (B) brain images of a dog with encephalopathy due to PSS. Note the hyperintense lesions in the lentiform nuclei evident on the T1-weighted images. (Torisu et al., 2005. Reproduced with permission from Wiley.)734 Animals with HMD may be difficult, if not impossible, to diagnose without a liver biopsy. These patients tend to have normal or slightly abnormal bloodwork abnormalities compared to PSS patients (e.g. serum albumin and cholesterol levels, mean corpuscular volume). Also, serum bile acid concentrations in HMD patients, especially postprandial, tend to be lower than in patients with PSS. Per-rectal scintigraphy results are also normal in animals with HMD. Other hepatic disorders also typically require liver biopsy for a diagnosis. Treatment for hepatic encephalopathy is directed at reducing the level of gut-derived toxins and controlling seizures, if present. A diet with a low amount of high-quality protein (e.g. K/D diet) is usually instituted to minimize ammonia production by gut bacteria. Oral medications such as lactulose, a synthetic disaccharide, with or without oral antibiotics (e.g. aminoglycosides), are administered, to decrease colonic bacterial production of volatile fatty acids and other potentially neurotoxic substances. Lactulose is hydrolyzed by colonic bacteria to produce organic acids (e.g. lactic, acetic, formic acid) and carbon dioxide. The organic acids lower the pH of the colon, trapping colonic ammonia in the form of nonabsorbable ammonium. Lactulose may also lead to a favorable alteration of the colonic flora, accelerated intestinal transit time, and may exert some antiendotoxin activity. Lactulose is administered at an initial dose of 0.5–1.0 ml/kg body weight, every 8 hrs. The dose is adjusted, if necessary, to result in two to three soft stools per day. Neomycin sulfate is poorly absorbed when administered orally, but is active against urea-splitting bacteria in the colon. The dose of neomycin is 20 mg/kg body weight, every 8 hrs. Oral ampicillin (22 mg/kg body weight, q 8 hrs) and metronidazole (7.5 mg/kg body weight, q 8 hrs) are also commonly administered to hepatic encephalopathy patients, for their effects on colonic flora, and as protection against sepsis. In severely encephalopathic patients, dilute betadine, and/or lactulose enemas have been recommended. Because of its caustic nature, the author prefers not to use betadine enemas in these patients. A lactulose enema is prepared using three parts lactulose to seven parts water. The enema is administered at a dose of 20 ml/kg and retained in the colon for 15–20 min. This is repeated every 4–6 hrs, if necessary. Some patients with hepatic insufficiency may be hypoglycemic and need supplemental parenteral glucose. It is important to prevent or reverse conditions that may exacerbate hepatic encephalopathy, such as alkalosis, hypokalemia, and gastrointestinal hemorrhage. In cases of postsurgical PSS intractable seizure activity, the author believes that brain swelling should be assumed, and mannitol therapy should be instituted. In addition, although bromide is a sound anticonvulsant choice in the PSS dog (no hepatic metabolism), it does contribute to the often obtunded mental state in these patients. The author has had success using intravenous levetiracetam (also no hepatic metabolism) in a limited number of PSS dogs with seizure activity. Anticonvulsant medication needs to be tapered to the individual patient, and generally administered at lower-than-standard dosages if metabolized by the liver. Treatment of the underlying disorder in hepatic encephalopathy cases is the key to controlling signs of neurologic dysfunction. In HMD and most acquired hepatic disorders, medical management involving dietary modification, lactulose, and other medications (e.g. prednisone, anticholestatic, and antifibrotic drugs for chronic active hepatitis) form the basis of treatment. For PSS, surgical attenuation of the shunting vessel(s) is usually recommended. Shunt attenuation using a device called an ameroid constrictor is commonly practiced; the ameroid constrictor allows for the progressive attenuation of the shunting vessel following placement. Some PSS patients will develop refractory generalized seizures after shunt ligation/attenuation, which can be fatal. This phenomenon has been suspected to be due, in part, to an abrupt decrease in brain benzodiazepine-like substances following shunt ligation; however, this phenomenon likely involves a more complicated constellation of neurochemical aberrations. Clinical signs of hepatic encephalopathy can often be reversed with appropriate medical therapy. The prognosis for each patient depends on the specific underlying disease responsible for the encephalopathy. Most extrahepatic PSS dogs are successfully treated surgically with partial or complete shunt attenuation. In addition to the ameroid constrictor, cellophane banding and intravascular thrombogenic coils have also been used in attempts to achieve a gradual closure of portosystemic shunts. Most recently, a percutaneously controlled hydraulic occluder device has been developed to provide the gradual postoperative occlusion of shunt vessels; preliminary data from this device are encouraging. In general, postoperative complications of PSS surgery are higher for cats than dogs, and surgical success rates are higher for dogs compared with cats with PSS. The prognosis for cats with extrahepatic PSS and both dogs and cats with intrahepatic PSS is guarded. HMD patients can be well controlled long term with medical therapy. The prognosis for dogs and cats with other hepatic disorders is highly variable. This category of metabolic encephalopathy encompasses uremic encephalopathy (UE), dialysis disequilibrium syndrome (DDS), and posttransplantation encephalopathy (PTE). Similar to hepatic encephalopathy, there are numerous proposed mechanisms to explain UE, which include the following: circulating neurotoxins, such as high levels of parathyroid hormone (PTH), which may have a direct toxic effect on neurons in addition to secondary effects from increased extracellular calcium levels; ionic imbalances, particularly hypercalcemia, which can lead to neuronal mineralization; hyperosmolality, causing cerebral neuronal dehydration; hypertension, which may lead to tortuosity, intimal proliferation, and necrosis of cerebral vasculature; uremic vasculitis affecting cerebral blood vessels; acid-base imbalance, specifically acidosis depressing cerebral function; and uremia-induced neurotransmitter imbalance in the brain. Low-grade anemia in some renal-failure patients may contribute to these aforementioned processes in producing encephalopathy. DDS is thought to be caused by an osmotic gradient between the brain and the extracellular fluid environment, due to overly rapid hemodialysis. The brain remains relatively hyperosmotic to the blood, perhaps due to the production of intraneuronal idiogenic osmoles during the uremic state. The gradient causes the neurons to imbibe water, leading to cerebral edema. In PTE, described in cats after renal transplantation, uncontrolled hypertension is thought to play a major causative role. Treatment of renal-associated encephalopathy depends primarily upon the management of the underlying kidney disease. Electrolyte and acid-base imbalances should be corrected, if indicated, and seizures should be controlled with anticonvulsant drugs. Managing arterial hypertension in cats before and after renal transplantation with propranolol, hydralazine, and/or acepromazine may help prevent the development of PTE. The prognosis of renal-associated encephalopathy is variable, and depends mainly on the specific renal abnormality. In most cases, the encephalopathy can be ameliorated or resolved with the control of uremia. The development of PTE is best avoided, as these cats tend to have severe signs of neurologic dysfunction, and a high mortality rate. The brain has an absolute requirement for glucose. Glucose enters the brain via a noninsulin-dependent facilitated transport mechanism. This transport mechanism requires a minimum blood glucose level to operate effectively. There are limited glycogen stores in the brain, and the neuronal energy depletion associated with severe (usually less than 45 mg/dl) hypoglycemia often results in clinical signs of encephalopathy. There are multiple causes of hypoglycemia, including overproduction of endogenous insulin or insulin-like substances (e.g. insulin-like growth factors) by pancreatic insulinomas or other neoplasms, glycogen depletion in neonatal/juvenile puppies (usually of toy/miniature breeds) and kittens, sepsis, and exogenous insulin overdose (i.e. diabetes mellitus patients, especially feline). Other less-common causes of clinically significant hypoglycemia include hypoadrenocorticism, liver failure, glycogen storage diseases, and the so-called hunting dog hypoglycemia syndrome, the latter of which may represent the combined effects of strenuous exercise with inadequate caloric intake. Imbalances of sodium (Na+) and calcium (Ca++) may cause clinical signs of encephalopathy, the severity of which tends to correlate directly with the rapidity of development of the particular imbalance. There are numerous potential causes for these electrolyte disturbances. Hypernatremia and hyponatremia are, for all practical purposes, synonymous with hyperosmolality and hypo-osmolality, respectively. Hypernatremia can lead to shrinkage of brain parenchymal cells. A potential secondary effect of this parenchymal shrinkage is stretching and tearing of small intracranial blood vessels with resultant hemorrhage. Both intracellular dehydration and intracranial hemorrhage may contribute to brain dysfunction in the hypernatremic state. With chronic hypernatremia (more than two or three days), brain parenchymal cells will produce osmotically active intracellular substances, called idiogenic osmoles, in an attempt to compensate for the increased extracellular osmolality. Hyponatremia can result in the swelling of brain parenchymal cells with subsequent brain edema. Brain parenchymal cells will compensate for chronic hyponatremia (more than two or three days) by actively extruding osmotically active intracellular components, such as potassium and amino acids. Overly rapid correction of either a chronic hypernatremic or a hyponatremic state may lead to severe encephalopathic signs. In the former scenario, the encephalopathy is due to brain edema (associated with the idiogenic osmoles), whereas in the second, it is likely due to axonal shrinkage (due to the relative lack of intracellular osmolality) and subsequent demyelination in the brain stem (particularly the thalamus), similar to central pontine myelinolysis in people. Hypercalcemia can cause decreased excitability of neuronal cell membranes, as well as direct toxic damage to neuronal intracellular energy-producing systems. Hypocalcemia may lead to increased excitability of neuronal cell membranes, as well as abnormal neurotransmission. Figure 7.29 Transaxial T2-weighted brain MR image, demonstrating lesions associated with central myelinolysis after rapid correction of hyponatremia in a dog. (O’Brien et al., 2004. Reproduced with permission from Wiley.)521 Treatment of EAE involves correction of the electrolyte disturbance, as well as investigation and potential treatment of the underlying cause for the electrolyte abnormality. Correction of hypernatremia is achieved by administering fluids that are hypo-osmolar to the patient. This can be achieved via using 5% dextrose (basically free water), half-strength or normal saline, depending upon the degree and chronicity of the hypernatremia. The amount of water to administer can be calculated according to the following formula: With relatively acute hypernatremia, the deficit can be corrected quickly using 5% dextrose. With chronic hypernatremia, the deficit should be corrected gradually over 48–72 hrs, starting with half-strength or normal saline, eventually switching to 5% dextrose. The chronically hypernatremic patient’s sodium level should not be lowered faster than 0.5 mEq/L/hr. Hyponatremia is corrected by administering sodium-containing fluids such as normal or hypertonic saline (in acute, severe cases). The amount of sodium to be replenished can be calculated as follows: As with chronic hypernatremia, chronic hyponatremia should be corrected slowly over 48–72 hrs, raising the patient’s sodium level by no more than 0.5 mEq/L/hr. Hypocalcemia is corrected in the emergency situation by slow intravenous infusion of 10% calcium gluconate at a dosage of 5–15 mg/kg over 10–30 min; this should work out to be 0.5–1.5 ml/kg. It is extremely important not to confuse the two ways of expressing dosage of this drug, as overdosage can be fatal. While administering calcium gluconate, the patient’s electrocardiogram (ECG) should be continuously recorded. The infusion should be stopped if premature ventricular contractions, shortening of the QT interval, or bradycardia are observed. Once the patient is stabilized, maintenance oral calcium and vitamin D supplementation can be initiated. Emergency therapy of the hypercalcemic patient usually involves diuresis with 0.9% saline (two to three times maintenance fluid rate) and furosemide (2–4 mg/kg intravenously, q 8–12 hrs, or a 5 mg/kg IV bolus, followed by 5 mg/kg/hr continuous infusion). Other therapies may include glucocorticoids and calcitonin administration. The prognosis for reversing encephalopathic signs due to electrolyte disturbances is generally favorable. The overall prognosis for the individual patient is highly variable and depends upon the specific disease process responsible for the electrolyte aberration. Elevated levels of serum and CSF lactate and pyruvate are characteristic features in people with Leigh’s syndrome; increased CSF levels of lactate and pyruvate were documented in the Australian Cattle dog puppies. With the exception of one Australian Cattle dog with a mild pleocytosis and elevated protein concentration, CSF evaluation has been normal in affected dogs. Advanced imaging (CT, MRI) has been performed in a limited number of dogs. Bilaterally symmetric, cavitary lesions in the brain and/or spinal cord were evident on imaging, which corresponded to lesions discovered at necropsy. Bilateral cavitary lesions were evident on CT imaging in the thalamus and medulla of the Yorkshire Terriers; electron microscopy of these lesions revealed abnormally shaped neuronal mitochondria. Similar to Leigh’s syndrome of humans, MRI lesions associated with mitochondrial encephalopathy in dogs are characteristically hypointense on T1-W images, hyperintense on T2-W images, and noncontrast enhancing (Fig. 7.30). Figure 7.30 T2-weighted transaxial (A) and dorsal (B) brain images from of an Alaskan Husky dog with mitochondrial encephalopathy. Note the bilaterally symmetric, hyperintense lesions in the thalamus. (Dr. J. Wakshlag, 2014. Reproduced with permission from Dr. J. Wakshlag.) Definitive diagnosis of these disorders is based on gross and histopathologic features of the CNS at necropsy. Bilaterally symmetric, spongiform/vacuolar lesions, primarily affecting gray matter, are the pathologic hallmark of these disorders. Histopathologically, multiple areas of the CNS are affected in ME dogs, but some regions are grossly abnormal on gross inspection. Spongiform brain-stem lesions from thalamus to medulla are prominent in Alaskan Huskies, the thalamic lesions being most conspicuous. Australian Cattle dogs tend to have cavitary lesions in cerebellar and brain-stem nuclei, as well as in both the cervical and lumbosacral intumescences. The English Springer Spaniel had similar lesions in the accessory olivary nuclei of the brain stem. Abnormal mitochondria in neurons and astrocytes have been demonstrated ultrastructurally in several cases of ME.
Encephalopathies: Disorders of the Brain
Introduction
Clinical signs of brain dysfunction (see also Chapters 2 through 4)
Disorders affecting the brain in dogs and cats (Table 7.1)
Degenerative
Lysosomal storage disease
Leukodystrophy/spongy degeneration
Neuronal vacuolation of Rottweilers and Boxer dogs
Multisystem neuronal degeneration/abiotrophy of Cocker Spaniels
Cognitive dysfunction syndrome (CDS)
Anomalous/Developmental
Congenital hydrocephalus
Caudal occipital malformation syndrome (COMS)
Intracranial arachnoid cyst (IAC)
Neuronal migration disorders
Dandy–Walker syndrome (DWS)
Miscellaneous malformations
Metabolic
Hepatic encephalopathy
Renal-associated encephalopathy
Hypoglycemic encephalopathy
Electrolyte-associated encephalopathy
Miscellaneous endocrine-related encephalopathies
Acid-base disturbance encephalopathy
Mitochondrial encephalopathy
Organic acidurias
Neoplastic
Primary brain tumors
Secondary brain tumors
Nutritional
Thiamine deficiency
Inflammatory/Infectious
Bacterial meningoencephalitis
Fungal meningoencephalitis
Viral meningoencephalitis
Protozoal meningoencephalitis
Rickettsial meningoencephalitis
Verminous meningoencephalitis
Miscellaneous infections meningoencephalitides
Granulomatous meningoencephalitis (GME)
Necrotizing meningoencephalitis of small-breed dogs
Eosinophilic meningoencephalitis
Hydrocephalus with periventricular encephalitis (HPE)
Ischemic/Vascular
Global ischemia
Thromboembolic disease (nonhemorrhagic/hemorrhagic infarcts)
Disease subgroup
Storage disease
Deficiency
Reported breeds
Glycoproteinoses
Fucosidosis
α-L-fucosidase
English Springer Spaniel
Mannosidosis
α-D-mannosidase
Domestic Shorthaired cat
Domestic Longhaired cat
Persian cat
Neuronal glycoproteinosis (Lafora’s disease)
Unknown
Basset Hound
Beagle
Poodle
Wire-haired Miniature Dachshund
Mixed-breed dog
Galactosialidosis
Protective protein/cathepsin A (protects lysosomal degradation of β-galactosidase and neuraminidase)
Schipperke
Oligosaccharidoses
Glycogenosis type Ia (von Gierke disease)
Glucose-6-phosphatase
Maltesea
Glycogenosis type II (Pompe’s disease)
α-glucosidase
Lapland dog
Domestic Shorthaired cat
Glycogenosis type IIIa
glycogen debranching enzyme (AGL gene)
Curly-Coated retriever
Glycogenosis type IV (Andersen’s disease)
glycogen debranching enzyme
Norwegian Forest cat
Sphingolipidoses
Gangliosidosis (GM1) type I (Norman-Landing disease)
β-galactosidase
Beagle crossb
English Springer Spaniel
Domestic Shorthaired cat
Siamese cat
Type II (Derry’s disease)
β-galactosidase
Alaskan Husky
Portuguese Water dog
Shiba dog
Korat cat
Domestic Shorthaired cat
Siamese cat
Gangliosidosis (GM2)
type I (Tay-Sachs’ disease)
Hexosaminidase A
German Shorthaired Pointer
Japanese Spaniel
Type II (Sandhoff’s disease)
Hexosaminidase A and B
Golden Retriever
Domestic Shorthaired cat
Korat cat
Glucocerebrosidosis (Gaucher’s disease)
β-D-Glucocerebrosidase
Sydney Silky Terrier
Globoid cell leukodystrophy (Krabbe’s disease)
β-D-Galactocerebrosidase
Basset Hound
Beagle
Blue Tick Hound
Cairn Terrier
Irish Setter
Miniature Poodle
Pomeranian
West Highland White Terrier
Domestic Shorthaired cat
Domestic Longhaired cat
Sphingomyelinosis (Niemann–Pick disease)
Type A
Sphingomyelinase
Miniature Poodle
Balinese cat
Siamese cat
Type B
Cholesterol esterification deficiency
Boxer dog
Domestic Shorthaired cat
Type C
NPC1 mutation (protein function unknown)
Domestic Shorthaired cat
Metachromatic leukodystrophy
Arylsulfatase A
Domestic Shorthaired cat
Mucopolysaccharidoses
Mucopolysaccharidosis type I (Hurler’s disease, Scheie disease, Hurler/Scheie disease)
α-L-iduronidase
Mixed-breed dog
Plott hound
Rottweiler
Domestic Shorthaired catc
Mucopolysaccharidosis type II (Hunter disease)
Iduronate-2-sulfatase
Labrador Retriever
Miniature Pinscher
Domestic Shorthaired cat
Siamese cat
Mucopolysaccharidosis type III A and B (Sanfilippo disease)
Heparan-N-sulfatase (type A)
N-acetyl-α-D-glucosaminidase (type B)
Dachshund (type A)
New Zealand Huntaway dog (type A)
Schipperke dog (type B)
Mucopolysaccharidosis type VI (Maroteaux-Lamy disease)
Arylsulfatase B
Chesapeake Bay Retriever
Miniature Pinscher
Miniature Schnauzer
Welsh Corgi
Domestic Shorthaired cat
Siamese cat
Mucopolysaccharidosis type VII (Sly disease)
β-D-glucuronidase
German Shepherd dog
Mixed-breed dog
Domestic shorthaired cat
Mucolipidosis II (I-cell disease)
N-acetylglucosamine-1- phosphotransferase
Domestic Shorthaired cats
Proteinoses
Ceroid lipofuscinosis (Batten’s disease)
Cathepsin Dd
American Bulldog
American Pit Bull Terriere
American Staffordshire Terriere
Australian Cattle dog
CLN5d
Border Collie
Chihuahua
Cocker Spaniel
Corgi
CLN2 (TTP1)d
Dachshund
Dalmatian
CLN8d
English Setter
Golden Retriever
Labrador Retriever
Miniature Schnauzer
Polish Owczarek Nizinny dog
Queensland Blue Heeler
Saluki
Terrier crossbreed
Tibetan Terrier
Yugoslavian Sheepdog
Domestic Shorthaired cat
Siamese cat
Signs: DISHAAL
Age first noticed
Score 0-3a
D: Disorientation/Confusion—Awareness, Spatial orientation
Gets stuck or cannot get around objects
Stares blankly at walls or floor
Decreased recognition of familiar people/pets
Goes to wrong side of door; walks into door/walls
Drops food/cannot find
Decreased response to auditory or visual stimuli
Increased reactivity to auditory or visual stimuli (barking)
I: Interactions—Social Relationships
Decreased interest in petting/avoids contact
Decreased greeting behavior
In need of constant contact, overdependent, “clingy”
Altered relationships other pets, less social/irritable/aggressive
Altered relationships with people, less social/irritable/aggressive
S: Sleep–Wake Cycles—Reversed Day/Night Schedule
Restless sleep/waking at nights
Increased daytime sleep
H: House Soiling (Learning and Memory)
Indoor elimination at sites previously trained
Decrease/loss of signaling
Goes outdoors, then returns indoors and eliminates
Elimination in crate or sleeping area
A: Activity—Increased/Repetitive
Pacing/wanders aimlessly
Snaps at air/licks air
Licking owners/household objects
Increased appetite (eats quicker or more food)
A: Activity—Apathy/Depressed
Decreased interest in food/treats
Decreased exploration/activity/play
Decreased self-care (hygiene)
A: Anxiety
Vocalization, restlessness/agitation
Anxiety, fear/phobia to auditory or visual stimuli
Anxiety, fear/phobia to places (surfaces, locations)
Anxiety/fear of people
Separation anxiety
L: Learning and Memory—Work, Tasks, Commands
Decreased ability to perform learned tasks, commands
Decreased responsiveness to familiar commands and tricks
Inability/slow to learn new tasks
Dog
Cat
Selegiline (CDS)
0.5–1 mg/kg sid in am
0.5–1 mg/kg sid in am
Propentofylline (CDS)
2.5–5 mg/kg bid
1/4 of a 50 mg tablet daily
Oxazepama
0.2–1 mg/kg sid–bid
0.2–0.5 mg/kg sid–bid
Clonazepama
0.1–1.0 mg/kg bid–tid
0.02–0.2 mg/kg sid–bid
Lorazepama
0.025–0.2 mg/kg sid–tid
0.025–0.05 mg/kg sid–bid
Diphendydraminea
2–4 mg/kg
1–4 mg/kg
Fluoxetine
1.0–2.0 mg/kg sid
0.5–1.5 mg/kg sid
Paroxetine
0.5–2 mg/kg
0.5–1.5 mg/kg
Sertraline
1–5 mg/kg sid or divided bid
0.5–1.5 mg/kg sid
Buspirone
0.5–2.0 mg/kg sid–tid
0.5–1 mg/kg bid
Trazodone
2–5 mg/kg (up to 8–10) prn–tid
Not determined
Phenobarbital
2.5–5 mg/kg bid
2.5 mg/kg bid
Memantine
0.3–1 mg/kg sid
Not determined
Gabapentin
10–30 mg/kg q 8–12 h
5–10 mg/kg q 12 h
Pregabalin
2–4 mg/kg q 8–12 h
1–2 mg/kg q 12 h
Ingredients
Dose
Senilife
Phosphatidylserine, Gingko biloba, vitamin B6 (pyridoxine), vitamin E, resveratrol
Dogs and cats (see label)
Aktivait
Phosphatidylserine, omega-3 fatty acids, vitamins E and C, L-carnitine, alpha-lipoic acid, coenzyme Q, selenium
Note: no alpha-lipoic acid in feline version
Separate dog and cat products
See label
Novifit
S-Adenosyl-L-methionine disulfate tosylate (SAMe)
Dog: 10–20 mg/kg sid
Cat: 100 mg sid
Neutricks
Apoaequorin
Dogs: 1 tablet per 18 kg
Prescription diet b/d Canine aging and alertness
Flavonoids and carotenoids from fruits and vegetables, vitamin E, vitamin C, beta-carotene, selenium, L-carnitine, alpha-lipoic acid, omega-3 fatty acids
Dogs
Purina One Vibrant Maturity 7 + Senior
Medium chain triglycerides (from coconut oil)
Dogs
Melatonin
Endogenous-based peptide
Dogs: 3–9 mg
Cats: 1.5–6 mg
Anxitane
Suntheanine
Dogs: 2.5–5 mg/kg bid
Cats: 25 mg bid
Harmonease
Magnolia and phellodendron
Dogs: up to 22 kg 1/2 tablet daily; > 22 kg
1 tablet daily
Cats: N/A
Zylkène
Alpha-casozepine
Dogs: 15–30 mg/kg/d
Cats: 15 mg/kg/d
Pheromones
Adaptil collar, diffuser, or spray for dogs
Feliway spray or diffuser for cats
As per label
Lavender
Aromatherapy for dogs
As per label
Drug
Dosage
Prednisone
0.25–0.5 mg/kg PO, q 12 hrs
Furosemide
0.5–4.0 mg/kg PO, q 12–24 hrs
Acetazolamide
10 mg/kg PO, q 6–8 hrs
Omeprazole
10 mg q 24 hrs (dogs less than 20 kg); 20 mg q 24 hrs (dogs more than 20 kg) PO
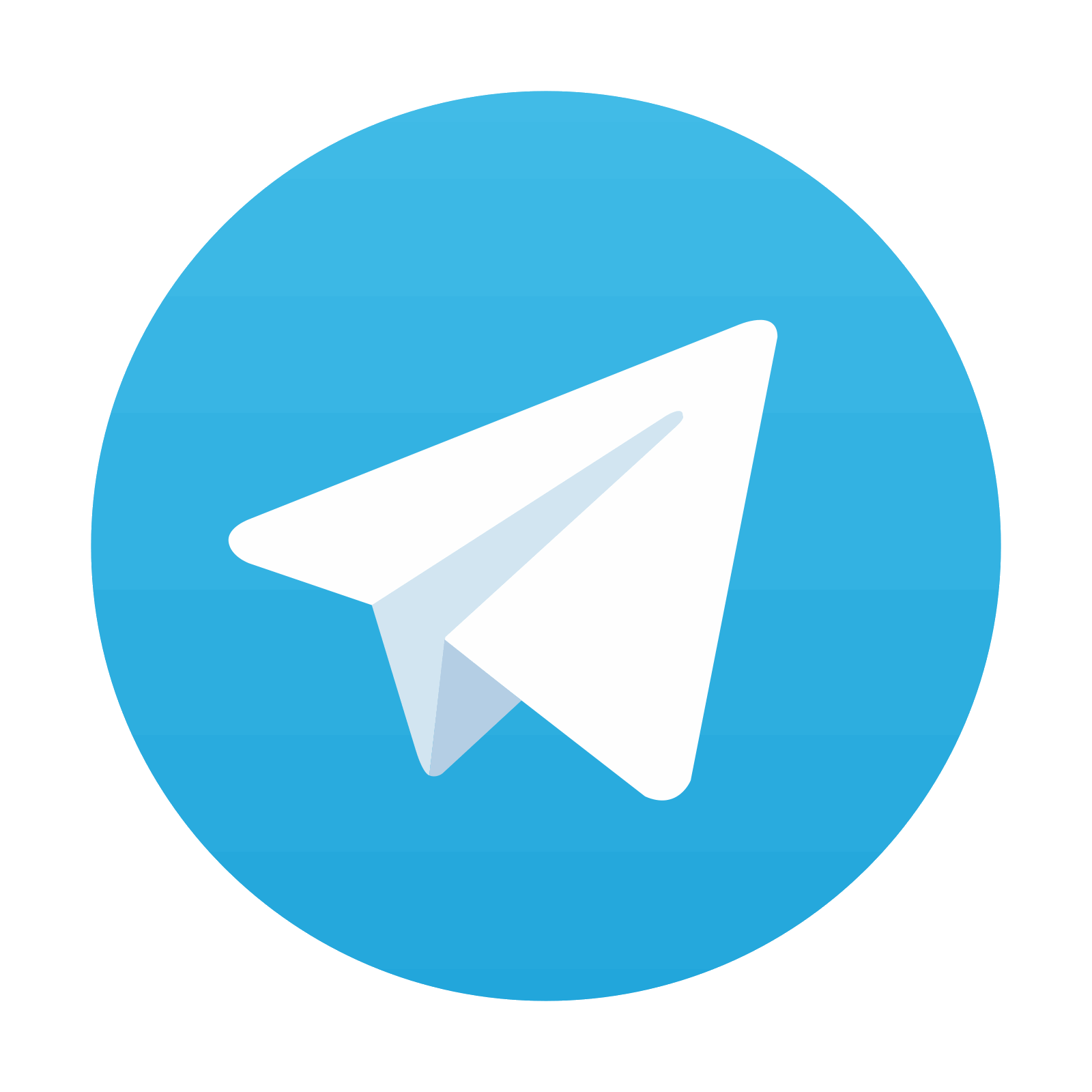
Stay updated, free articles. Join our Telegram channel
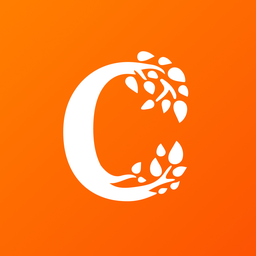
Full access? Get Clinical Tree
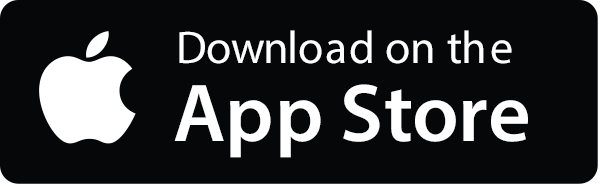
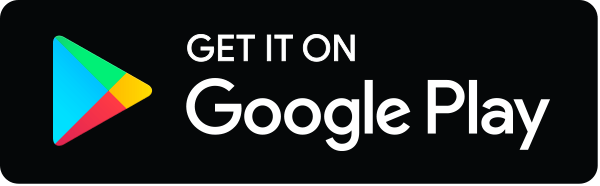