30 Margarethe Hoenig Diabetes mellitus has been recognized for centuries as a debilitating disease, characterized by the excretion of “sweet urine” (mellituria), polydipsia, wasting of tissue, and the development of ketoacidosis, hyperosmolar coma, and death. The role of the pancreas in the development of diabetes was first recognized in 1886 when Minkowski and von Mering produced diabetes by total pancreatectomy in the dog. In 1921, Banting and Best extracted the active compound from pancreas and showed that it was able to control hyperglycemia in diabetic dogs and humans. Insulin was prepared for the first time in crystalline form in 1926 by Abel, and it took 34 more years until its amino acid sequence was demonstrated by Sanger. Finally, in 1963 insulin was synthesized by Meyenhofer and coworkers, and in 1964 by Katsoyannis and coworkers. In 1967, Steiner discovered that insulin was synthesized as a larger precursor, proinsulin. An even larger molecule, named preproinsulin, was identified as a precursor for proinsulin in 1976 by Chan and coworkers (for a detailed review see Bliss, 1983). These research efforts have culminated in the biosynthesis of human insulin by recombinant DNA techniques, allowing large-scale production of the hormone in 1983 (Frank and Chance, 1983; Johnson, 1983). Spontaneous diabetes has been reported in many species. Diabetes is a major health problem and one of the leading causes of death in humans. In animals, it occurs most frequently in the dog and cat, with an incidence of approximately 0.5–1%. Overt diabetes is rare in horses, cattle, and sheep, although obese horses may develop metabolic syndrome, which might include abnormal glucose concentrations. Isolated cases of diabetes have been reported in mules, ferrets, pigs, newts, buffalos, monkeys, and fish (Stogdale, 1986). Diabetes also has been reported in several breeds of birds (Stogdale, 1986). Diabetes in humans has been classified into two major categories, which are of interest to veterinary medicine: type 1 diabetes, previously called insulin-dependent diabetes mellitus or juvenile-onset diabetes, and type 2 diabetes, previously called noninsulin-dependent diabetes mellitus or maturity-onset diabetes. Based on glucose-tolerance testing (Kaneko et al., 1977; O’Brien et al., 1985), it has been determined that most diabetic dogs and cats are insulin deficient. Insulin is produced in the beta cells of the islets of Langerhans in the endocrine pancreas. The islet also contains glucagon-secreting alpha cells, somatostatin-secreting delta cells and PP or F cells, which secrete pancreatic polypeptide. The beta cells compose about 60–80% of the islet. The endocrine cells are arranged in a nonrandom distribution, with beta cells forming a central core surrounded by a mantle of the other three cell types in some animals and humans (Bonner-Weir and Orci, 1982). In the cat, the beta cells are located in the periphery (O’Brien et al., 1986), while in the horse, the alpha cells form a central core (Helmstaedter et al., 1976) surrounded by beta cells. Insulin exists initially as preproinsulin, which is cleaved to proinsulin in the endoplasmic reticulum. Proinsulin is a large polypeptide consisting of an A and B chain and a connecting peptide (Figure 30.1). By proteolytic cleavage, four basic amino acids and the connecting peptide are removed, and proinsulin is converted to insulin (Steiner et al., 1969). This reduces the molecular weight from 9000 (proinsulin) to one of 6000 (insulin) and one of 3000 (C-peptide). This conversion occurs soon after proinsulin is transported to the Golgi complex, where it is packaged into secretory granules. These granules therefore contain an equimolar amount of insulin and C-peptide. In the secretory granules, insulin is complexed with zinc and stored. Figure 30.1 Proinsulin. The amino acid sequence of insulin shows little species variation. Human insulin differs from porcine and rabbit insulin by a single amino acid, whereas dog insulin is identical to porcine insulin. Bovine insulin differs from human insulin by three amino acids and from feline insulin by one amino acid (Neubauer and Schoene, 1978; Porte and Halter, 1981; Hoenig et al., 2006a). Antiserum directed against insulin of one species usually cross-reacts with insulin of other species. Antiinsulin serum generally also cross-reacts with heterologous proinsulin, while C-peptide has no immunoreactivity against insulin antiserum. The biological activity of proinsulin, however, is much less than that of insulin. Feline proinsulin has been cloned and expressed (Hoenig et al., 2006a). The secretion of insulin from the beta cell occurs through the process of exocytosis in which there is fusion of the secretory granule with the plasma membrane. Insulin secretion is highly regulated primarily by glucose, but also by other fuels, hormones, and neurotransmitters (Porte and Halter, 1981). On a cellular level, insulin release stimulated by glucose and other fuels is thought to be initiated by closure of ATP (adenosine triphosphate)-dependent K channels, which leads to depolarization of the beta cell and influx of calcium into the cytosol through voltage-gated calcium channels (Arkhammar et al., 1987; Cook et al., 1988). The increase in cytosolic free calcium triggers pulsatile insulin secretion. In addition, augmentation of this response occurs by both a K+-ATP channel-independent Ca2+-dependent pathway and K+-ATP channel-independent Ca2+-independent pathways of glucose action (Henquin, 2011). The pathway that is Ca2+ dependent but independent of ATP-K channels is called the amplification pathway. The exact mechanism is not understood (for review see Straub and Sharp, 2002). Insulin is released from the beta cell in a pulsatile fashion. It is thought that these rapid oscillations are generated through intraislet mechanisms, because they can be seen even in isolated islets and beta cells (Berman et al., 1993). Pulsatile secretion becomes abnormal in diabetes; however, pulsatility can be restored by beta cell rest (Laedtke et al., 2000). In both type 1 and type 2 diabetes, islet function and insulin secretion are abnormal. It is now clear that in both types a loss of beta-cell mass has occurred and that the fundamental difference between the two major types of diabetes lies in the etiology of the beta-cell dysfunction and its severity but not its presence or absence (Porte, 1991). It is controversial whether a reduction in beta cell mass or function play the major role in the pathogenesis of type 2 diabetes (for review see Meier and Bonadonna, 2013). It appears that both occur together and are tightly related. Insulin resistance, frequently seen with obesity in humans and cats (Kahn et al., 2006; Hoenig et al., 2006b) is an important concomitant factor in type 2 diabetes and puts an additional stress on the beta cell. However, the insulin resistance of obesity alone is not sufficient to cause diabetes. This is shown by the fact that over 80% of obese people are not diabetic; a similar assessment has not been made in cats. It is not known how many obese cats actually become diabetic. Obesity increases the risk of diabetes; however, most obese cats have normal glucose control because they counteract peripheral insulin resistance with a decrease in endogenous glucose output by the liver (Kley et al., 2009; Hoenig et al., 2011, 2012, 2013). It is interesting that, while the prevalence of obesity in people and cats is about equal, about 8% of humans develop type 2 diabetes, whereas the prevalence of diabetes in cats is <1%. The reason for this discrepancy is not known. Evidence suggests that disordered insulin secretion may contribute to development of insulin resistance and hence represent an initiating factor in the progression to type 2 diabetes (Schofield and Sutherland, 2012). An early marker of beta cell dysfunction is a loss of pulsatility of insulin secretion. This leads to hepatic insulin resistance and higher uncleaved proinsulin concentrations (for review see Wahren et al., 2012). An early marker is also a change in the proinsulin/insulin secretion ratio (Haffner et al., 1997). In cats and humans, among a few others, the increase in insulin release from a reduced beta-cell mass in type 2 diabetes is associated with release and deposition of islet amyloid polypeptide, leading to further loss of beta cells (Porte and Kahn, 1989; Hoenig et al., 2000a). It is thought that the majority of dogs have a form of diabetes similar to type 1 diabetes in people, that is involving a genetic component, which is influenced by environmental input. The possibility of a genetic basis for canine diabetes has been suggested by the occurrence of the disease in dog families, and the presence of DLA haplotypes that seem to confer risk of diabetes. In type 1 diabetes in people, the loss of beta cells is caused primarily by immune-mediated destruction (MacLaren et al., 1989). Beta-cell antibodies are present in approximately 50% of naïve diabetic dogs (Hoenig and Dawe, 1992). In later studies, 4/30 dogs were found to be positive for antibodies against the 65-kDa isoform of canine glutamic acid decarboxylase and 3/30 also had antibodies against the insulinoma-associated antigen-2 (IA-2), both frequently found in human type 1 diabetics (Davison et al., 2008a). In another study, 5/40 newly diagnosed dogs had antibodies against insulin (Davison et al., 2008b). Beta-cell antibodies have not been demonstrated in diabetic cats (Hoenig et al., 2000b). Insulin secretion can also become autonomous, resulting in hyperinsulinemia associated with hypoglycemia. Insulin-secreting tumors (adenomas or carcinomas; also called insulinomas) occur infrequently and have been described in the dog, cat, ferret, cattle, and a pony (Capen, 1990). The diagnosis of an insulinoma can be difficult, but frequently it can be made on the basis of an inappropriately high serum insulin concentration. In humans, the diagnosis is often made based on high proinsulin and/or C-peptide concentrations (Chammas et al., 2003). Insulin is the most potent physiological anabolic agent. In insulin-responsive tissues, it facilitates cellular uptake and metabolism of glucose. Insulin promotes the synthesis of glycogen, protein, and fat and is involved in the uptake of ions such as K into the cell. Insulin exerts its effect by binding to specific receptors on the plasma membrane of cells. The insulin receptor is a tetrameric structure consisting of two subunits: an alpha and a beta subunit. Two of each of these subunits are joined together via disulfide bonds. Both subunits are glycosylated and exposed to the extracellular milieu; only the beta subunit is exposed to the intracellular environment. The insulin receptor has tyrosine kinase activity. Binding of insulin leads to autophosphorylation of the receptor and these phosphorylated Tyr residues serve as docking sites for downstream effectors (Le Roith and Zick, 2001; Schinner et al., 2005). Once the insulin receptor kinase is activated, the presence of insulin is not necessary for its continued activity. The receptor is inactivated by dephosphorylation. Despite our knowledge of insulin–receptor interaction, our understanding of molecular events that link the insulin–receptor to the regulation of cellular metabolism is poor. Some, but not all, of the actions are due to the phosphorylation or dephosphorylation of target proteins on serine and threonine residues. The mediation of the mitogenic responses involves the mitogen-activated protein (MAP) kinase pathway, whereas the metabolic responses of insulin, such as glucose transport, involve phosphatidyl-inositol-3 (PI3)-kinase. Diabetes has also been linked to alterations in the function of both endoplasmic reticulum (Kaneto et al., 2005) as well as mitochondria (Lowell and Shulman, 2005). The existence of a relatively specific insulin-degrading enzyme (IDE, insulinase, insulin-specific protease) has been reported by several investigators (for a review see Duckworth, 1990). Insulin-degrading activity has been found in essentially all tissues examined, and high activity has been seen in liver, kidney, muscle, brain, fibroblasts, and red blood cells. The enzyme seems to be located predominantly in the cytoplasm but can be found in other subcellular compartments. Plasma membrane preparations also contain this enzyme. Some receptor-bound insulin is degraded on the membrane, while some insulin is released intact from the receptor, and some is internalized with the receptor, and insulin degradation is then initiated in endosomes. The degradation of insulin via this process is rapid. Other enzymes may also be involved in the degradation of insulin, and their role and factors controlling insulin-degrading activity in various tissues and disease states needs to be examined. The biological activity of insulin preparations is documented using a bioassay that is based on the capacity of insulin to lower blood glucose concentrations. All but one insulin preparation used in human medicine have been standardized at 100 U/ml; a U 500 (500 U/ml) regular insulin preparation is still available from Eli Lilly. All insulin preparations used for humans are of recombinant origin, either containing the exact amino acid sequence of human insulin, or mutations thereof. This has led to a variety of new insulins with different action times. Regular human insulin, lispro, glulisine, and aspart can be given intravenously. There are two insulins that have been approved for veterinary use: ProZinc® is a human recombinant protamine zinc insulin, and Vetsulin® is a pork zinc insulin and is currently the only animal-derived insulin still available on the US market. Both are approved for use in cats; Vetsulin in addition is for use in dogs. Both of these insulins contain 40 U/ml and are administered subcutaneously. For correct dosage, insulin preparations are administered with syringes that are calibrated for the appropriate standardization (e.g., U 100 syringes, U 40 syringes). All commercially available insulins in the United States contain no more than 25 parts per million of proinsulin. The insulin preparations currently available are summarized in Table 30.1. Table 30.1 Insulin preparations Based on action profiles, insulin preparations can be divided into fast, intermediate, and long acting. Generally, lispro and aspart, as well as the regular insulin preparations, have a quick onset of action (15-30 minutes) and short duration, whereas the NPH (neutral protamine Hagedorn), glargine, detemir, protamine zinc, and the pork zinc insulin preparations have a longer onset and duration of action. There is, however, considerable species and individual variation in the insulin action profiles. It is important for the practitioner to realize that the time course of action of any insulin preparation may not only vary considerably between different individuals, but also in the same individual from day to day (Hoenig and Ferguson, 1991; Church, 1981). It is also important to realize that the pharmacokinetics change with different doses. The time periods listed by manufacturers should only be considered as initial guidelines. Individual responses to insulin and the “fine-tuning” of insulin therapy have to be assessed by measuring blood glucose concentrations at frequent intervals, yielding what is called a “glucose curve”. In order to decrease stress effects, glucose monitoring also can be performed by measuring interstitial glucose concentrations using a continuous glucose monitoring system (e.g., Guardian Real-Time; Medtronic). Of the newer short-acting insulin preparations, lispro has been used successfully for the treatment of ketoacidotic dogs (Sears et al., 2012). There are currently no reports of the clinical use of aspart in pets. The pharmacokinetics of insulin aspart are similar to those of regular insulin when given intravenously in people. The plasma half-life is 11 minutes with insulin aspart and 12 minutes with regular insulin. When given subcutaneously, there is also little difference between the two insulins. However, both show great individual variations in plasma concentrations (Plum et al., 2000). Glargine and detemir have been used extensively in diabetic cats (Weaver et al., 2006; Roomp and Rand, 2009; Roomp and Rand, 2012) and also in dogs (Sako et al., 2011; Fracassi et al., 2012; Hess and Drobatz, 2013). Although labeled as “long-acting” insulin preparations, they usually need to be given twice daily for optimal glucose control. The dosage of detemir is generally less than that of other insulin preparations. Insulin, thus far, needs to be injected for the effective treatment of diabetes. The short-acting insulin preparations can be injected intravenously, intramuscularly, and subcutaneously; the intermediate and long-acting insulin preparations are for subcutaneous injection. The preferred injection site is the flank for those injections. Some of the intermediate and long-acting insulin preparations, but not detemir and glargine, are suspensions and need to be gently mixed before administration. It is recommended to shake pork zinc insulin vigorously before the first use. The shelf life of most insulin preparations is 28 days when stored at room temperature. If refrigerated, unopened bottles last until the expiration date of the vial. Attempts have been ongoing to produce insulins that do not require injection. Exubera®, which was produced by Pfizer, Sanofi-Aventis, and Nektar Therapeutics, was a rapid-acting, dry-powder type of insulin that was delivered to the lungs via a special bong-shaped inhaler device, and received FDA approval in 2006. It was withdrawn from the market in 2007 because of weak sales. Oral-lyn® has been developed by Generex. It is a novel oral (buccal) insulin spray product, which is delivered into the human mouth by way of the company’s proprietary RapidMist drug delivery system. It completed phase III clinical trials in India in 2013 and is awaiting approval by the Indian authorities. It remains to be seen if such a preparation could be used in pets. Insulin deficiency is characterized by increased catabolism. The breakdown of glycogen, increased endogenous glucose output, and decreased utilization of glucose in peripheral tissues, especially muscle and adipose tissue, leads to hyperglycemia. Protein breakdown fuels gluconeogenesis, and fat breakdown leads to increased fatty acids in blood and their transfer primarily to the liver, where they are used for energy, ketone body or very low-density lipoprotein (VLDL) production. They can also be reesterified leading to fatty liver. Treatment of diabetes must be adjusted to the clinical presentation of the animal. Usually, two broad forms can be differentiated: uncomplicated and complicated diabetes mellitus. For practical purposes, complicated diabetics are those that cannot take food orally, such as the vomiting ketoacidotic patient or the comatose hyperosmolar patient (Hoenig and Ferguson, 1991). The goal of treatment of a diabetic dog is to maintain blood glucose concentrations in a high normal to mildly hyperglycemic range for most of the day to alleviate the biochemical effects and clinical signs of insulin deficiency and avoid hypoglycemia. In cats, the treatment regimens are controversial. Some clinicians advise to maintain blood glucose concentrations in a normal to mild hyperglycemic state, similar to what is recommended for diabetic dogs; that is, the blood glucose should stay between 5 and 15 mmol/l (90-270 mg/dl) for most of the day (Reusch, 2010), and the nadir should be between 90 mg/dl and 160 mg/dl, avoiding the dangers of hypoglycemia in a patient that cannot convey the more subtle signs of low blood glucose. Others have proposed an insulin treatment regimen even more rigorous than what is desirable in humans (Roomp and Rand, 2012), and advocated to achieve glucose concentrations in diabetic cats between 2.8 and 5.5 mmol/l (50 and 100 mg/dl), that is a range where cats may experience biochemical as well as clinical hypoglycemia. The goal of this regimen is to increase remission rates. While these investigators have reported higher remission rates than others, without blinding the studies and providing detailed information about the health status of the patients entering those studies and the feeding protocols, a comparison between the success of a particular treatment regimen is not possible. Furthermore, the dangers of hypoglycemia cannot be ignored and might compromise the quality of life of both owner and pet. The fast-acting insulin preparations are used for the complicated diabetic patient. These animals can be treated with low-dose constant rate insulin infusions (see Table 30.2 for treatment protocol) or intramuscular insulin injections with regular insulin or lispro insulin using 0.1 units/kg as needed to keep blood glucose concentrations at approximately 200 mg/dl. Glargine, a long-acting insulin preparation, has also been used for the treatment of ketoacidosis in cat and was given intramuscularly as well as subcutaneously (Marshall et al., 2013). Because of glargine’s long duration; however, such a protocol would eliminate the ability to quickly intervene. Table 30.2 Low-dose, continuous IV infusion protocol for the treatment of diabetic ketoacidosis. Source: Modified from Macintire, 1993 and Sears et al., 2012.
Drugs Influencing Glucose Metabolism
Insulin
History
Chemistry and Biosynthesis
Secretion
Mechanism of Action
Metabolism
Preparations and Properties
Trademark
Common name
Strength
Administration
Company
Humalog®
Lispro
U100
External pump,
Eli Lily
Novolog®
Aspart
U100
Intravenous,
Novo Nordisk
Apidra®
Glulisine
U100
subcutaneous
Sanofi-Aventis
Humulin® R
Regular human insulin,
U100
External pump,
Eli Lily
Humulin® R
recombinant DNA origin
U 500
intravenous
intramuscular
subcutaneous
Humulin® N
NPH human insulin, recombinant DNA origin
U100
Subcutaneous
Prozinc®
Protamine zinc human recombinant insulin, DNA origin
U40
Boehringer-Ingelheim
Vetsulin®
Pork zinc insulin
U40
Merck Animal Health
Lantus® (Glargine)
Recombinant human insulin analog, DNA origin
U100
Sanofi-Aventis
Levemir® (Detemir) Detemir
Recombinant human insulin analog, DNA origin
U100
Novo Nordisk
Tresiba® (Insulin Degludec)
Recombinant human insulin analog, DNA origin
U200
Therapeutic Uses
Treatment of Diabetes Mellitus in Dogs and Cats
1. Place intravenous catheter.
2. Start fluid therapy (0.9% NaCl or Normosol R; consider replacement and maintenance fluid requirements, as well as supplementation with K and/or P as needed). Rehydrate the animal.
3. [Insert urinary catheter and attach to a closed monitoring system.]
4. Add regular insulin or lispro insulin (2.2 units/kg/day) to 250 ml of saline solution. Use pediatric infusion set or infusion pump for accurate administration. Use protocol shown below.
5. Monitor blood glucose concentrations every 1 hour.
6. Monitor urinary output.
7. Monitor electrolytes every 8 hours. Adjust if necessary.
8. Change saline solution to 2.5% dextrose in 0.45% saline, when blood glucose reaches approximately 250 mg/dl (13.8 mmol/l). Continue to administer insulin. Supplement K and/or P as needed. Adjust fluid rate and glucose concentration of the fluid as follows:
Blood glucose (mg/dl)
IV fluids
Insulin rate (ml/hour)
>250
0.9% saline
10
200–250
0.9% saline 2.5% dextrose
7
150–200
0.9% saline 2.5% dextrose
5
100–150
0.9% saline 5% dextrose
5
<100
0.9% saline 5% dextrose
stop
9. Keep animals on this regimen until they are able to eat without vomiting.
10. Stop insulin infusion in the evening. Start treatment of the uncomplicated diabetic the next morning.
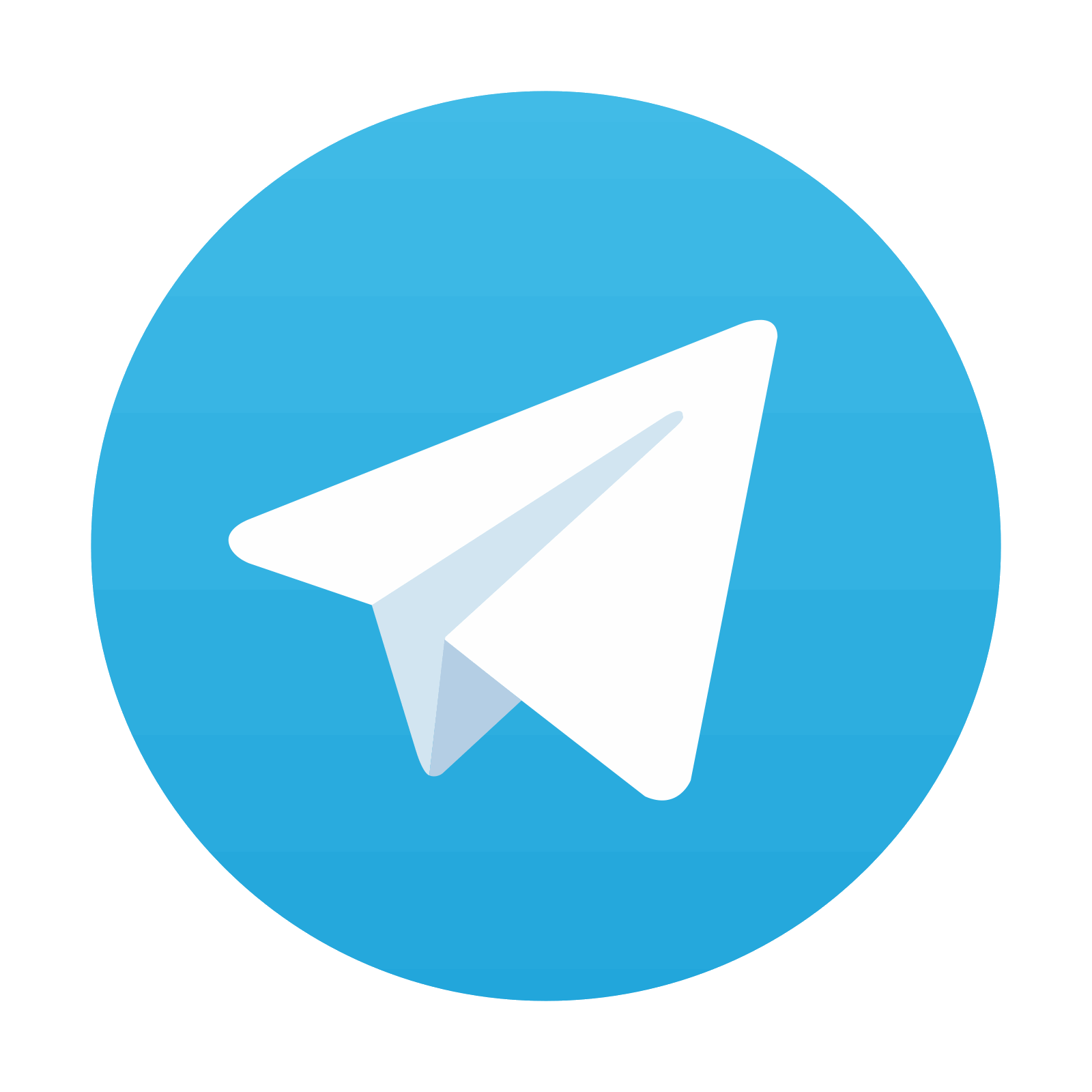
Stay updated, free articles. Join our Telegram channel
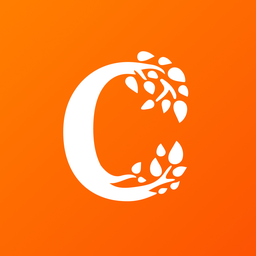
Full access? Get Clinical Tree
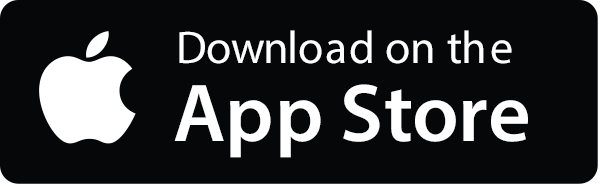
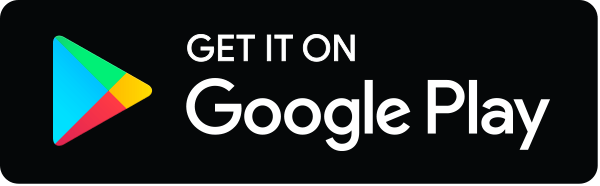