Adeline N. Boettcher and Christopher K. Tuggle Department of Animal Science, Iowa State University, Ames, IA, USA Within the last two decades, there has been a steady rise in the number of swine biomedical models available for researchers to use to address different research questions related to cancer. As research has indicated that swine may be more translational to humans than mice, there has been an increased prevalence of the development of these models for certain biomedical questions. Recent estimates show that nearly 90% of cancer drugs that were preclinically tested in mice fail in humans once they enter clinical trials (Mak et al. 2014; Uhl and Warner 2015). The drug development process – from idea conception to FDA approval – can cost anywhere from $1 to $2 billion dollars (Van Norman 2016; DiMasi et al. 2016). Testing in an inappropriate animal model could lead to a time and financial burden that could otherwise be mitigated if other, more translational animal models are developed. The field of preclinical immunotherapy is continually advancing, with new mouse models being developed and validated for different research questions. There are numerous humanized and xenograft models, and subsequent techniques, that have already been designed in mice. Knowledge gained from these mouse studies can be adapted for use in swine models. Pigs may even be able to overcome some of the challenges that are encountered in mouse models, including difficulties in establishing certain types of human patient‐derived xenograft (PDX) tissues (Jackson and Thomas 2017). Within the last decade numerous swine models have been described for different types of cancer (Schook et al. 2015; Principe et al. 2018; Clark et al. 2015; Schachtschneider et al. 2017; Kalla et al. 2020), human tissue and cell engraftment (Boettcher et al. 2020; Singer et al. 2019; Boettcher et al. 2019a), stem cell research (Lee et al. 2014), and infectious disease research (Rajao et al. 2017). Herein, a review of the swine models that are used in current cancer research are described. First, the two main models that have been developed for cancer research – pigs with severe combined immunodeficiency (SCID) and pigs with inducible mutations in TP53 and KRAS – will be described. Next, a description of the different cancer models that have been developed in swine will be discussed. Cancer models such as breast, hepatocellular carcinoma (HCC), and gliomas have all been initiated in swine and are continuing to be developed. Cancer models for other anatomical areas will be discussed within this chapter as well. Next, hypotheses will be proposed and questions that are likely to be addressed within these new emerging pig models will be proposed. Lastly, SCID pig care will be discussed, as these animals require special facilities and attention. Together, the topics within this chapter will cover a wide range of disciplines that can be built upon for the use of pig models for cancer research specifically related to immunotherapies. There has been increased interest in generating different genetic swine models for biomedical research. Generation of these new models has been facilitated by different methods of genetic modification that have been developed for use in pigs. This section provides an overview of different swine genetic models that have either been naturally discovered or genetically engineered that can be used as a tool for immunotherapy and cancer research. SCID is defined as the lack of an adaptive immune system. SCID mice were first discovered in the early 1980s and have since been developed as an invaluable tool for research due to their ability to accept human xenografts (Bosma et al. 1983; Mosier et al. 1988; McCune et al. 1988; Rongvaux et al. 2014; Wunderlich et al. 2018). Thus, development of a SCID pig model would prove useful in cancer immunotherapy research. The first SCID pigs were serendipitously discovered in a viral challenge study (Basel et al. 2012; Ozuna et al. 2013; Ewen et al. 2014). A small fraction of the group of assumed‐to‐be wildtype piglets died unexpectantly in a viral challenge experiment, and upon necropsy and subsequent blood and histological analysis, the piglets were found to lack a thymus and serum antibodies. Upon further genetic characterization and breeding of prospective carriers in this population, two different mutant ARTEMIS (ART) alleles (termed ART12 and ART16) were discovered that cause SCID in these pigs (Waide et al. 2015). The Artemis protein is required for proper variable (V), diversity (D), and joining (J) recombination of T‐ and B‐cell receptor expression, and subsequent T‐ and B‐cell development (Ma et al. 2002). The original piglets from the viral challenge study were discovered to be ART12/ART16 compound heterozygotes. Both alleles also cause SCID in the homozygous state as well (ART SCID pigs will be referred to as ART−/− herein). (Powell et al. 2017). The ART−/− SCID pigs have a T− B− NK+ cellular phenotype (Waide et al. 2015) and NK cells are functional in vitro (Powell et al. 2016). Early studies showed that these pigs were capable of accepting xenografts of human tumor cell lines (Basel et al. 2012). These pigs have already been used in a variety of research fields including investigating innate‐adaptive interactions during influenza infection (Rajao et al. 2017), human skin engraftment (Singer et al. 2019), and establishment of human ovarian cancer xenografts (Boettcher et al. 2019a). Recombination activating genes 1 and 2 (RAG1/2) are also involved in the process of variable, diversity, and joining (VDJ) recombination (Oettinger et al. 1990; Schwarz et al. 1996; Mombaerts et al. 1992). Knock‐out of RAG1/2 causes T− B− NK+ SCID in humans and mice (Schwarz et al. 1996; Mombaerts et al. 1992), and thus researchers have developed RAG1 and RAG2 knock‐out SCID pigs. RAG1/2 proteins are required for VDJ recombination needed for T‐ and B‐cell development. Both RAG1 and RAG2 knockout pigs have been generated through methods using TALENs or gene targeting methods (Lee et al. 2014; Huang et al. 2014; Ito et al. 2014; Suzuki et al. 2016; Chen et al. 2019). Similar to ART−/− pigs, RAG1−/−, and RAG2−/− have a T− B− NK+ cellular phenotype (Lee et al. 2014; Huang et al. 2014; Ito et al. 2014; Seida and Suzuki 2017). RAG knockout pigs have been shown to engraft human induced pluripotent cell lines (Lee et al. 2014; Choi et al. 2017), and showed some differences in engraftment outcomes with equivalent SCID mice. IL2RG is the common subunit in several heterodimer interleukin receptor proteins that are required for proper signaling for IL‐2, IL‐15, IL‐4, IL‐7, and IL‐21, several of which are involved in the development of T, B, and NK cells (Sugamura et al. 1996; Matthews et al. 1995; Lin and Leonard 2018). IL2RG has been knocked out in pigs using various techniques such as gene targeting (Suzuki et al. 2012), zinc finger nucleases (Watanabe et al. 2013), as well as CRISPR/Cas9 (Boettcher et al. 2020; Chen et al. 2019; Kang et al. 2016). IL2RG knock‐out pigs have a T− B+ NK− cellular phenotype, with non‐functional B cells due to a lack of T‐helper cells (Suzuki et al. 2012; Watanabe et al. 2013; Kang et al. 2016; Hara et al. 2018). Mouse models that have a T− B− NK− cellular phenotype are more permissible to human xenograft growth success compared to T− B− NK+ models. NK cells in mice have shown to contribute to human xenograft rejection (Christianson et al. 1996; McDermott et al. 2010), thus a pig model without functional NK cells would be an optimal model for human xenograft studies. The ART−/− (Waide et al. 2015), RAG1−/− (Huang et al. 2014; Ito et al. 2014), and RAG2−/− (Lee et al. 2014; Suzuki et al. 2016) mutant pigs still have NK cells that could potentially be functional in vivo, depending on the genetic background (Powell et al. 2016). Therefore, different research groups have worked toward generating pigs that have mutations in both genes in the VDJ recombination pathway (ART or RAG1/2) and IL2RG to generate pigs that have a T− B− NK− cellular phenotype. Double knockout RAG2−/− IL2RG−/Y pigs were the first double mutant pig model described (Lei et al. 2016). These pigs displayed a T− B− NK+/− cellular phenotype. Some NK cells were present; however, it was hypothesized that these cells developed due to hypomorphic mutations with IL2RG and were not functional. These pigs were developed as a model to study viral infection in an immunocompromised host (Lei et al. 2016). Recently, the development and initial characterization of an ART−/− IL2RG−/Y pigs has been described. To generate these pigs, CRISPR/Cas9 site‐directed mutagenesis was used to introduce a mutation within the IL2RG gene in the ART−/− model described earlier (Boettcher et al. 2020). These pigs lacked T, B, and NK cells in blood and lymphoid organs. When ART−/− IL2RG−/Y fetuses were injected with human hematopoietic stem cells in utero, human T cells developed within these pigs (Boettcher et al. 2020; Boettcher et al. 2019b). Further details of humanized SCID pigs are discussed later within this chapter. TP53 and KRAS are commonly mutated in many human cancers (The ICGC/TCGA Pan‐Cancer Analysis of Whole Genomes Consortium 2019); therefore, generation of a pig with inducible mutations in either of these genes would prove useful for the development of new cancer systems in a large animal model. A transgenic pig model, termed Oncopig, was created that encoded Cre recombinase inducible KRASG12D and TP53R167H mutant gene expression (Schook et al. 2015). Cells isolated from Oncopigs that are exposed to Cre recombinase (by Adeno‐Cre, an adenovirus carrying Cre gene) become tumorigenic. Additionally, intramuscular injection of Cre within muscle tissue also cause tumors to develop in vivo (Schook et al. 2015). Later in the chapter, further discussion on the different cancer models that are being developed from the Oncopig model will be discussed. Other pig models that have been created are pigs with an inducible TP53R167H mutant gene (Sieren et al. 2014; Leuchs et al. 2012). CRISPR/Cas9 (Tanihara et al. 2018) and TALENs (Shen et al. 2017) have also been used to knock out TP53 function. An inducible KRASG12D model has also been developed for cancer research as well (Li et al. 2015). The benefit of mutant TP53 and KRAS pigs is that they all have an intact immune system, and thus do not require as much attention to care as the SCID pigs (described later in this chapter). Thus, these pigs can easily be transported back and forth between imaging facilities for longer‐term studies to track tumor growth and/or response to therapy. These pigs have previously been successfully imaged using CT, MRI, and ultrasound (Schook et al. 2015; Sieren et al. 2014; Leuchs et al. 2012) as is done with human patients for cancer diagnosis and monitoring. Prior to clinical testing, it is critical to have a validated animal model with the specific type of cancer being investigated. In recent years there has been an expansion in cancer types that are available to study in swine. These cancer models consist of both swine cancers, as well as xenografted human cancers in immunodeficient pigs. This section will give an overview of the different cancer types that have currently been described within pig models. One early study of B‐cell lymphoma in swine described the development of nine tumor cell lines (Cho et al. 2007). Two cell lines, chronic myelogenous leukemia 14736 and posttransplant lymphoproliferative disease 13271, from this original study were transduced with a GFP expressing lentivirus, which would allow for B‐cell lymphoma tracking in vivo (Cho et al. 2007; Schenk et al. 2019). The two established swine cell lines were passaged through NOD SCID gamma (NSG) mice to select for aggressive tumor variants (Cho et al. 2007). The cell lines were derived from a line of pigs with a fixed major histocompatibility complex (MHC) background, which can facilitate pig‐to‐pig transplant studies due to the absence of immune rejection issues (Sachs et al. 1976). GFP+ B‐cell lymphoma cells were injected into MHC matched swine and were detectable in peripheral blood for up to 48 hours post injection (Schenk et al. 2019). In another B‐cell lymphoma model, a porcine lymphoblastic cell line was derived from a sub‐line of the Massachusetts General Hospital MHC‐defined miniature swine (Andrews et al. 2019). This cell line was isolated from a spontaneous malignancy from a 2.5‐year‐old pig. Whole blood was collected, and the cells were isolated and cultured to expand and purify the tumor cells. The cells were cultured, passaged, and propagated in NSG mice (Andrews et al. 2019). There were three main cell lines that were characterized in this study (21353.1B2, 21353.F28, and 21353.2F8‐23482) that were immunophenotyped by flow cytometry. B‐cell lymphoma cell lines were CD3− CD172− CD16− CD25+ CD45RA+ CD79α+ with MHC class I and II expression. The cell lines also expressed Igμ and Igλ (Andrews et al. 2019). Both in vivo and in vitro models of breast cancer have been developed in swine (Clark et al. 2015; Rowson‐Hodel et al. 2015). In one study, primary porcine breast epithelial cells were isolated and immortalized by transfection with an SV40 LT expression vector. These cell lines then underwent a second set of transfections with a miRNA to known down porcine BRCA1 expression (Clark et al. 2015). Characterization of this cell line revealed that knockdown of BRCA1 resulted in altered cell morphology and increased growth rate. Additionally, the cells were further characterized for their expression of cancer stem cell markers EpCAM and ALDH1, which were both highly expressed in BRCA1 knockout cells. Development of a tissue‐specific BRCA1 knockout is warranted to further develop an in vivo porcine model (Clark et al. 2015). Additionally, these cells could be transplanted into pigs with a matched MHC genetic background for further model development. In another model of breast cancer in swine, porcine mammary epithelial cells (pMECs) were subjected to lentiviral plasmid transduction or infection using either human elongation factor 1α (EF1α)‐tdTomato or human phosophoglycerate kinase‐tdTomato plasmids in both in vitro and in vivo models (Rowson‐Hodel et al. 2015). This study assessed the feasibility of generating swine breast cancer cell lines, organoids, and in vivo breast tumors. In their in vitro studies, pMECs were isolated from freshly collected tissue and subjected to transduction with either of the plasmids. Cells were injected into NSG mice subcutaneously or into the mammary fat pads with either hydrogel or Matrigel. Tumors began developing between 4 and 16 weeks post injection. In the in vivo experiments, EF1α‐tdTomato lentivirus was injected into the mammary gland ducts of female pigs. Within 10 days post injection, western blots of the tissues showed expression of EF1α‐tdTomato (Rowson‐Hodel et al. 2015). This model could be developed for future in vivo testing. The adenomatous polyposis coli (APC) tumor suppressor gene plays an important role in the progression of colorectal cancer (Eshghifar et al. 2017). A pig model has been developed with a stop signal at codon 1311 in the APC gene (APC1311) (Flisikowska et al. 2017; Sikorska et al. 2018). APC1311 pigs develop polyps within the colon and rectum. Immunohistochemical evaluation of these polyps showed similarities to human colorectal cancers. Additionally, CD3+, CD4+, and CD8+ cells were present within the tumor microenvironment (Flisikowska et al. 2017), which suggests that this model could be used within protocols on immunotherapeutic testing for colorectal cancer. Glioblastomas are a unique model to develop in swine, as researchers can take advantage of the blood–brain barrier to introduce human cells into immunocompetent animals. In one early study, immunocompetent Landrace pigs underwent intracerebral injections with human U87MG glioma cell line (Selek et al. 2014). Pigs were immunosuppressed with cyclosporin to help prevent any potential rejection of injected cells. After approximately 30 days post injection, neurological symptoms, including seizures and apathy, began to occur. Tumors were visualized with MRI over time to monitor tumor growth, and then were collected and assessed by histopathology. Tumors had increased cellularity and were characteristic of neurological tumors (Selek et al. 2014). In another study, Yucatan minipigs were used instead (Khoshnevis et al. 2017). These pigs underwent immunosuppression with cyclosporine as well. Human U87 cells were injected through the skull in the pigs. CT imaging was used to assess the growth of tumors over time. In a study of nine injected pigs, four of the pigs developed tumors of 3000 mm3 in size by day 14 post injection. Histopathological assessment of the tumors showed that tissues were highly cellular and stained positively for vimentin (Khoshnevis et al. 2017). A model of HCC in swine has been developed from the Oncopig genetic background that express oncogenic TP53R167H and KRASG12D (Schachtschneider et al. 2017). To generate the HCC cell line, Oncopig primary hepatocytes were isolated and infected with Adeno‐Cre for the induction of TP53R167H and KRASG12D mutations. Genetic analyses were performed to determine the expression of drug metabolism and hepatocyte functional genes in the swine HCC cells to compare to human HCC cells; similar expression profiles were reported. Histopathological assessment was also performed on the porcine HCC cells. The cellular phenotype resembled human HCC by cellular morphology and expression of high levels of cytokeratin and vimentin. The porcine HCC cells were autologously transplanted into an Oncopig and a tumor was detected at four days post cell inoculation. Histopathological assessment of the developed tumor had infiltration of CD3+ T‐lymphocytes, suggesting that this porcine model would be a potential candidate for immunotherapy testing (Schachtschneider et al. 2017). Human hepatocytes have been introduced into the liver of pre‐immune swine during gestation (Fisher et al. 2013), which could help with reconstructing a faithful human tumor microenvironment within pigs. Continued development of sophisticated swine models could involve humanized livers to help resemble the human liver microenvironment. Early in the discovery of the naturally occurring ART−/− SCID pigs, one of the early research questions that was assessed was if these pigs could accept human xenografts. Human melanoma cells (A375SM) were injected into the ears of SCID pigs and tumors developed within six days post injection (Basel et al. 2012). A sentinel lymph node biopsy model has also been reported using a RAG2−/−
9
Development of Swine Models for Cancer Research: SCID Pigs and Other Emerging Pig Cancer Models
9.1 The Emergence of New Swine Biomedical Models
9.2 Overview of Existing Swine Biomedical Models
9.2.1 ARTEMIS Deficient SCID Pigs
9.2.2 RAG1 and RAG2 Knockout SCID Pigs
9.2.3 IL2RG Knockout SCID Pigs
9.2.4 Double Mutant SCID Pigs
9.2.5 Inducible TP53 and KRAS Mutant Pigs
9.3 Overview of Existing and Emerging Cancer Models in Pigs
9.3.1 B‐Cell Lymphoma
9.3.2 Breast Cancer
9.3.3 Colorectal Carcinoma
9.3.4 Glioblastoma
9.3.5 Hepatocellular Carcinoma
9.3.6 Melanoma and Histiocytoma
Stay updated, free articles. Join our Telegram channel
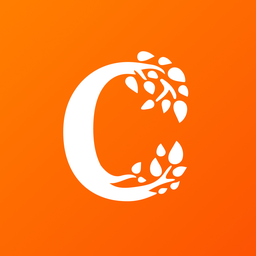
Full access? Get Clinical Tree
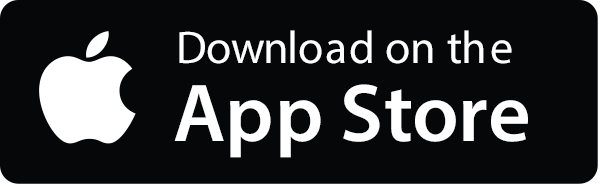
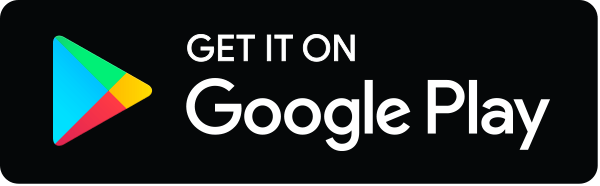