, Monika Hassel2 and Maura Grealy3
(1)
Centre of Organismal Studies, University of Heidelberg, Heidelberg, Germany
(2)
Spezielle Zoologie, Universität Marburg FB Biologie, Marburg, Germany
(3)
Pharmacology and Therapeutics, National University of Ireland Galway, Galway, Ireland
5.1 Xenopus: Standard of Reference for Vertebrate Development
5.1.1 Amphibian Embryogenesis Is Textbook Prototype for Understanding Vertebrate Development
Amphibians represent the archetype of vertebrate development. Figure 5.1 quotes the phylogenetic positions of vertebrates that are often investigated in the laboratory. From the Ancient World (Aristotle, Box1.1) up to the eighteenth century incubated chick eggs were the gold standard for animal development; since 1900 amphibians took over this position. The modified development of reptiles, birds and mammals can be deduced readily from the amphibian model exemplified by frogs and newts. The amphibian egg is rich in yolk and large (often 1–2 mm in diameter), yet the egg is able to undergo holoblastic cleavage, and cleavage converts a typical blastula to a gastrula displaying textbook features. In addition, amphibian embryos develop outside the mother and are therefore accessible to experimentation at all stages. The transparent jelly coat can easily be removed, surgically or chemically (after fertilization the envelope can be removed with 2.5 % cysteine-HCl, pH7.4). Pieces cut out from an embryo are able to continue development in sterile salt solutions without added nutrients, thanks to a supply of yolk in each cell. The eggs are suitable for microsurgical operations, which can be performed by hand with glass needles prepared in the laboratory; no complicated and expensive apparatus is needed.
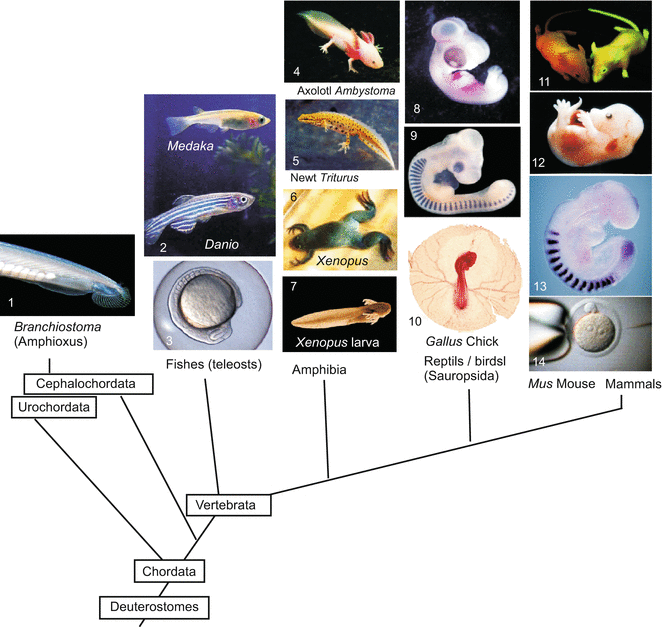
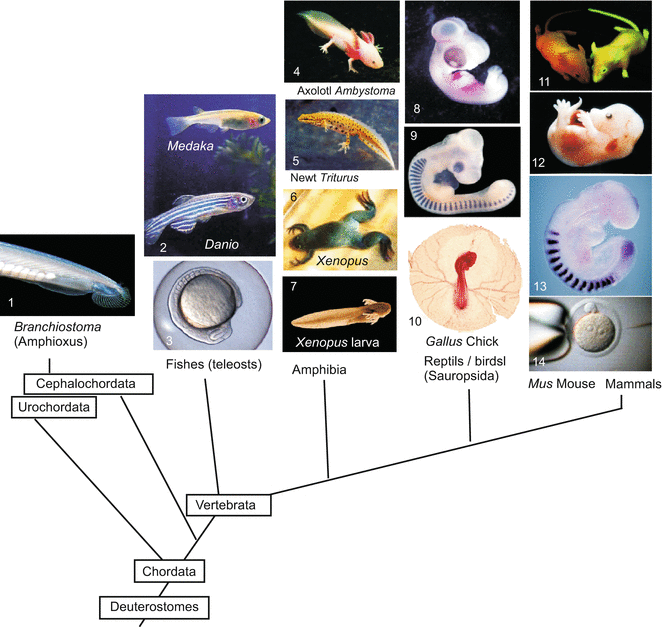
Fig. 5.1
Model organisms II. Vertebrates, that are dealt with or mentioned in this book, placed in the phylogenetic tree that is currently preferred on account of molecular genetic data. (The Cephalochordate Branchiostoma represents a side line of the Chordata that does not belong to the Vertebrata). Image copyrights belong to 1 Jonathan Veracaripe, 5 Julius Kramer, 11 ethlife, ETH-Zürich, 12 Michael F McElwaine, 13 Dr Alexander Aulehla, EMBL; Images reproduced with kind permission of the authors. Further pictures are part of the study collection of WM
At the beginning of experimental embryology eggs and embryos of frog specimens of the genus Rana ( R. temporaria in Europe, R. pipiens in America) were the preferred subjects that had to tolerate still rough surgical interventions. Under Hans Spemann, who was to win the Nobel Prize for his experimental studies with amphibians, embryos of newts (genus Triturus) became the principal actors in classic developmental biology. Between 1950 and 1970 the leading role was assigned to a new protagonist, the African clawed toad Xenopus laevis (Figs. 5.2 and 5.3). Although surgery may be more difficult with this species, it has many other favourable features. Xenopus always stays in water, is easily maintained, and because Xenopus is bred in the laboratory, there is no need to take endangered animals from natural populations (species conservation). In particular, Xenopus can be induced to spawn at any time by injection of chorionic gonadotropic hormones (Fig. 5.2). (Even human gonadotropin can be used; and injection of female urine to induce spawning in Xenopus was the first assay for pregnancy in human medicine.)
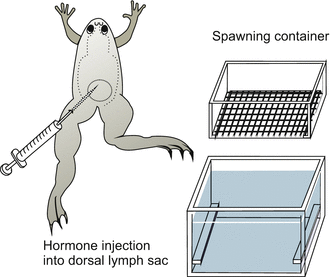
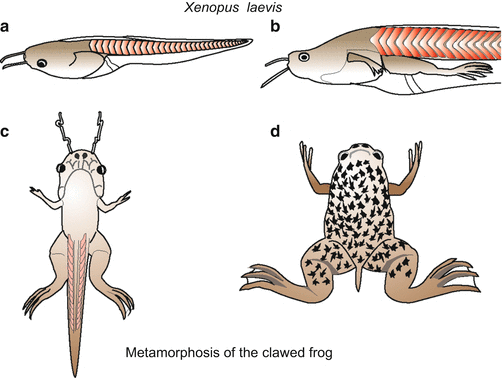
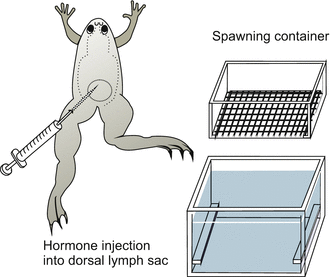
Fig. 5.2
Xenopus. Spawning container and injection of gonadotropic hormones to induce spawning
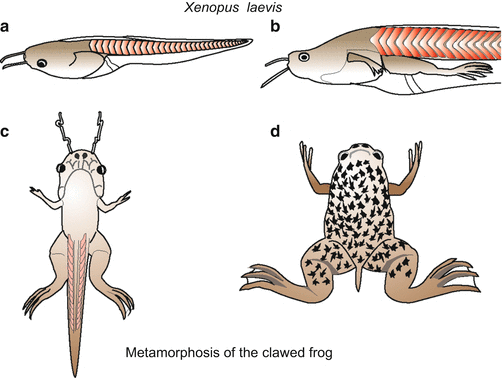
Fig. 5.3
Larval development and metamorphosis of the clawed frog Xenopus laevis
Xenopus laevis displays, however, an unwanted feature, too. The species is (pseudo)tetraploid. If one wished to generate homozygous mutations, not only two but four identical alleles had to be brought together in extensive and time-consuming cross-breeding programs. Practically, this is almost impossible. Therefore, recent genetic work is being focussed on the diploid sibling species Xenopus tropicalis. For this species methods have been developed to introduce transgenes, but these methods are amenable to specialists only.
Generally, experimental results are scored in embryos up to the tadpole stage. The larva of Xenopus (Fig 5.3) is transparent. It feeds by sweeping in planktonic algae and other microorganisms. As in other amphibians, the fundamental reorganization into the adult clawed toad is controlled by the hormones prolactin and thyroxine (Chap. 20).
5.1.2 The Study of Oogenesis in Xenopus Yielded Basic Knowledge, Applicable to Oogenesis Also in Other Vertebrates
Amphibian embryogenesis is the textbook prototype for understanding vertebrate development. One scarcely would understand oogenesis in humans if oogenesis in Xenopus had not been studied thoroughly already (Fig. 6.1). Embryos of Xenopus make extensive use of maternal gene transcripts stored in the oocyte.
In 1958 researchers at the University of Oxford discovered a mutant whose cells, although diploid, contained only one nucleolus in their nuclei instead of two nucleoli present in the wild type. Nucleoli are the factories where ribosomes are manufactured. These factories are constructed at two homologous chromosomes that contain 450 copies of the ribosomal genes (18S-, 5,8S-, 28S-rDNA) clustered in the nucleolus organizer region of the chromosomes. The heterozygous mutant, called 1-nu, was viable, because one set of ribosomal genes suffices to make enough ribosomes in oogenesis.
in the Mendelian ratios 1:2:1. The 0-nu/0-nu offspring who lacked both nucleoli were unable to produce ribosomes and, therefore, were not viable in the long term. However, surprisingly, embryonic development proceeded rather normally and the embryos even reached the stage of free-living tadpoles. Then they died.This surprising finding prompted the working hypothesis that oocytes might be provided with ribosomal RNA and the message for ribosomal protein even before they undergo meiosis. This would enable embryos arising from 0-nu eggs and 0-nu sperm to manufacture ribosomes without making use of their own genome, which lacks the corresponding genes. Perhaps, in the oocytes from which the 0-nu/0-nu embryos arose, message for the production of ribosomes was transcribed before meiosis led to the haploid stage. Before completion of meiosis in the oocytes of the 0-nu/1-nu mother, a normal chromosome was still present besides the mutant chromosome that was left after meiosis in the oocyte (while the normal chromosome was allocated to a polar body cell). The analysis of the phenomenon has led to a deeper understanding of oogenesis.
Crossing 1-nu × 1-nu resulted in homozygous 2-nu specimens (containing two nucleoli)
Heterozygous 1-nu and 0-nu specimens (one nucleolus) and
Homozygous 0-nu/0-nu individuals (no nucleolus)
In fact, amphibian oocytes have a supply enabling the embryo to pass through embryogenesis even without any contribution of its own genome. However, normal zygotic transcriptional activity commences in the stage called the ‘midblastula transition’ shortly before gastrulation begins (see as follows).
In amphibian oogenesis meiosis starts in the ovary of a young female soon after metamorphosis and takes several months. The meiotic prophase proceeds up to the diplotene stage. Then the prophase is interrupted for a long time during which the oocyte undergoes extensive growth. In the nuclei of the oocytes extensive transcriptional activity is initiated. As an expression of this high activity, lampbrush chromosomes, rDNA amplification, and multiple nucleoli occur in the nucleus of the oocyte (Figs. 8.4, 8.5 and 8.6). Furthermore, the liver of the female supplies maternal vitellogenins (protein) that are carried to the ovary by the blood stream, taken up by the oocyte and stored in the form of yolk granules. Oogenesis in vertebrates is described in more detail in the Chaps. 6 (human) and 8 (in general).
5.1.3 External Cues, Gravity and Sperm Provide Instructions for the Specification of the Body Axes and Bilateral Symmetry
Even before cleavage starts, processes are initiated which lead to the establishment of the egg’s spatial coordinates – that is to the establishment of bilateral symmetry. Unlike the egg of Drosophila, in the amphibian egg the maternal stores do not completely predetermine the future bilateral body organization. In its outward appearance the Xenopus egg, and more so frog eggs, presents a dark (black) animal hemisphere and a white vegetal hemisphere. Besides this rotationally symmetrical pigmentation pattern, no other polarity axis is visible. Yet, being a bilaterally symmetrical organism with right and left sides, a belly and a back, the vertebrate animal needs two axes of asymmetry: an antero-posterior axis and a dorso-ventral axis. The dorso-ventral median plane separates mirror-image symmetrical body halves the axes of which point from the midline to the outside.
How are these axes established? What is the relationship between the animal–vegetal axis and the two final coordinate systems of the body?
If we view the egg as a globe of the earth, and project the future embryo onto this globe, the head of the embryo will come to lie near the animal ‘North Pole’ (in other amphibians it will lie on the Tropic of Cancer) and the head–tail line will extend along a longitudinal line over the Northern Hemisphere, cross the equator, and terminate in the Southern Hemisphere near the Tropic of Capricorn (Fig. 5.4). The general directive that specifies that the head should be situated in the Northern and the tail in the Southern Hemisphere is laid down in the internally visible organization of the egg (animal–vegetal asymmetry). However, the embryo needs a further instruction to determine along which longitude the line should extend. The specification of this head-tail line is called dorsalization, and is accomplished by the interaction of several events. Besides the given animal–vegetal egg axis, there is also:
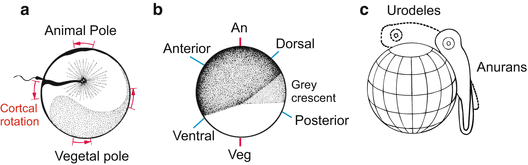
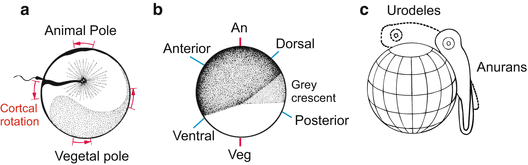
Fig. 5.4
Amphibian. Determination of bilateral symmetry. The future dorsal-posterior side (tail position) is diagonally opposite the entry point of the sperm. (a) After the sperm has entered, a sperm aster (black) appears in the centre of the egg, and cortical rotation commences. (b) Side view of the grey crescent which appears during cortical rotation. (c) The projection of the embryos onto the egg sphere does not take into account cell movements during gastrulation and, therefore, does not represent a true fate map. The projections merely superimpose the initial egg sphere and the finished tail bud larva
1.
the point where the sperm enters the egg, and
2.
the gravitational force of the (real) earth
The sperm can only attach on the animal hemisphere, but the exact point is determined by chance. After its entry, the ‘fertilization membrane’ is elevated, just as in the sea urchin egg. This elevation enables the egg to rotate so that the yolk-rich, heavy vegetal hemisphere points downward in response to gravity.
For dorsalization to occur, a uniform rotation of the entire, unaltered egg would not be much help. However, not all components of the egg move to the same extent and in the same direction. Induced by the sperm and influenced by gravity, active movements within the egg lead to an asymmetrical rearrangement of egg components. While the inner cytoplasm, heavy with dense yolk, sinks down and remains stabilized by gravity an active cortical rotation commences. Cortex in this context designates the mass-dense peripheral layer of cytoplasm associated with the cell membrane. The layer contains dense assembles of actin filaments and bundles of microtubules. Microtubules arranged parallel to the cell membrane are displaced against each other driven by kinesin motor proteins. The coordinated movement of the microtubules causes the egg membrane and outer cortical layer of the cytoplasm to shift relative to the inner cytoplasmic mass about 30° towards the point of sperm entry (Fig. 5.4). This rotation causes an asymmetrical rearrangement of egg components including maternal RNA. Plasma that in the unfertilized egg occupied the area around the vegetal pole is displaced to a site diagonally opposite the sperm entry point where the blastopore will form. Bilateral symmetry is now established; anterior and posterior, dorsal and ventral are defined.
The rotation of the cortical layer relative to the inner mass also causes a redistribution of pigment granules in the animal hemisphere. In eggs of the frog Rana the redistribution sometimes leads to a partial depigmentation on the future dorsal side opposite the point of sperm entry. The region of diminished pigment is referred to as the grey crescent. The grey crescent or its spatial equivalent (in Xenopus, a grey crescent is not visible) marks the region where gastrulation will be initiated and the blastopore will form. In Rana, the egg now has not only an invisible internal but also a visible bilaterally symmetrical organization: A line drawn from the grey crescent over the animal pole to the sperm entry point coincides with the line extending from the tail bud over the back to the head.
Such projections, however, must not be misunderstood as a definitive fate map. The material located between the animal pole and the grey crescent will largely be displaced into the interior during gastrulation. Cellularized egg material located around the grey crescent will shift into the head region, and material near the poles will come to lie in the tail region.
The cellular and molecular means by which sperm and gravity affect the molecular organization of the egg are partially known. The cytoplasmic rotation that occurs during fertilization leads to a segregation and repatterning of maternal cytoplasmic determinants, in particular an enrichment of components specifying the location of the Spemann organizer (see Sect. 5.1.10).
5.1.4 Cleavage and Gastrulation Come into the Picture as Textbook Prototypes; During Gastrulation Cell Material Is Incorporated into the Interior Giving Origin First to the Archenteron and Subsequently to the Mesoderm
Radial, holoblastic cleavage leads to a blastula (Fig. 5.5 illustrates real cleavage, Fig. 5.6 a didactically idealized cleavage). The subsequent gastrulation combines processes of invagination, involution, and epiboly. It starts in the centre of the area corresponding to the grey crescent in frogs. A group of cells sinks into the embryo, forming a groove and pulling along adjoining cells: the blastopore (primitive mouth, first mouth) is formed. The crescent-shaped groove of the blastopore becomes elongated and eventually forms a circle (Fig. 5.7). The dorsal sector of the circle is referred to as the upper blastopore lip which in the world of developmental biologist earned some fame (Sect. 5.1.10). The cells constituting the lip are constantly changing, because they are travellers merely passing through. Another picture that illustrates the dynamic structure of the lip is a waterfall: apparently constant when viewed from distance, constantly changing at a closer look.
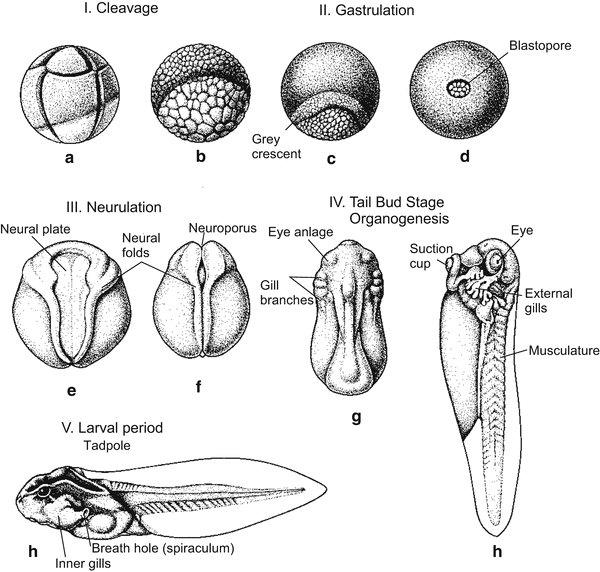
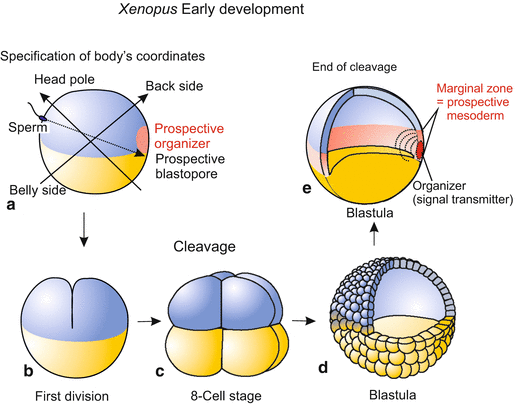
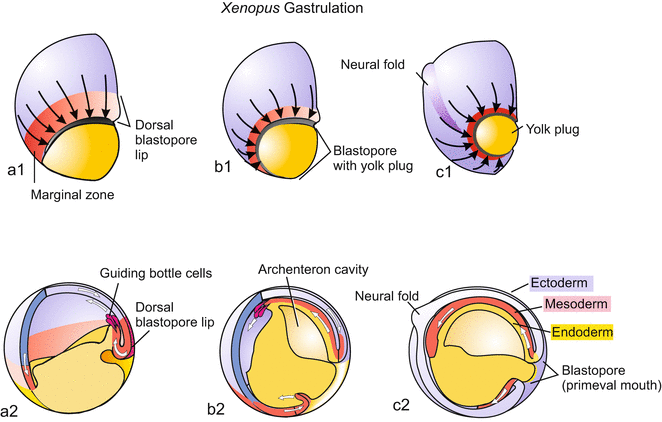
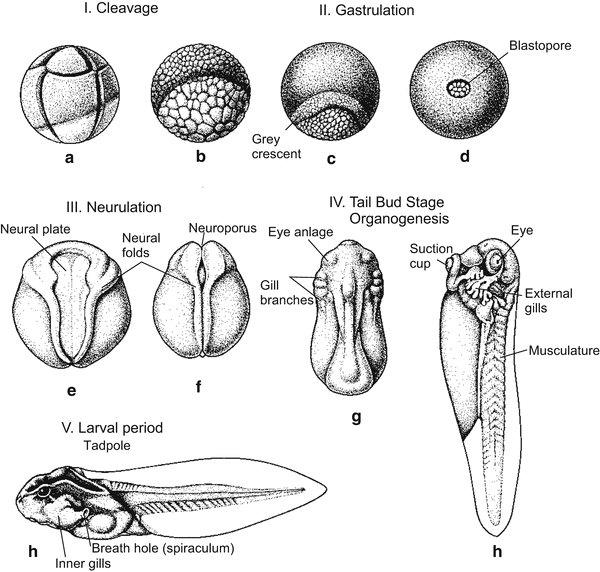
Fig. 5.5
Embryo development of a frog Rana (after Ch Houillon). (c) The grey crescent lies above the slit-shaped blastopore
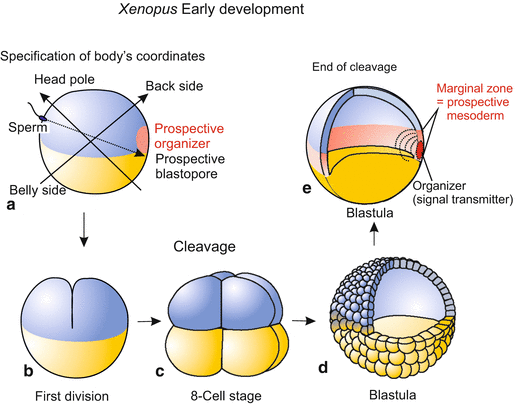
Fig. 5.6
(a–e) Amphibian. (a) Polarity axes in the fertilized egg (zygote); (b–e) Cleavage; (e) Blastula at the beginning of gastrulation
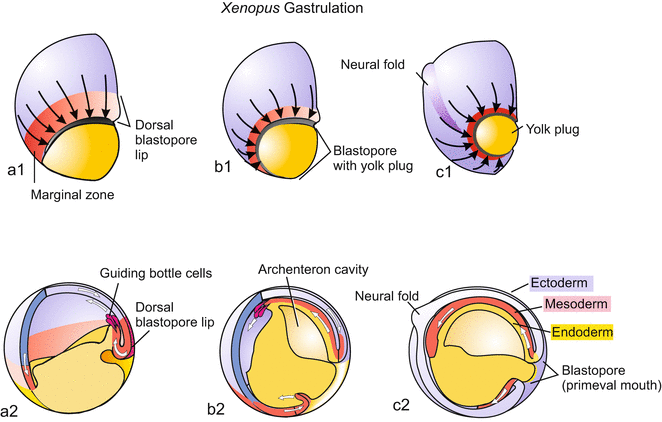
Fig. 5.7
(a–c) Amphibian. Gastrulation. (a1–c1) View from outside onto the blastopore; (a2–c2) Embryo dissected, lateral view
In traditional terminology one speaks of invagination when the crescent area buckles inward to form the primitive mouth and its edges (lips). Attracted by signals emanating from the region around the blastopore, cells of the blastoderm stream together towards the blastopore (convergence movement); arriving at the blastopore, they roll over the lip and squeeze into the interior. The turning around the lip is called involution.
Once inside the embryo, the cells move in an anterior direction as a sheet and spread along the entire inside surface of the blastoderm, thereby forming the vesicular archenteron (primary intestine). Involution and gliding are partly the result of changes in cell adhesiveness and are supported by the formation of lobopodia by the cells located at the leading edge. These leading and pioneering cells are known as bottleneck cells due to their shape (Figs. 5.7a2 and 14.1). Guided by these cells the invaded cell cohorts move as coherent sheet along the inner surface of the blastoderm forming the wall of the archenteron.
This active involution is supported by epiboly of the outer blastoderm: Although more than half of the blastula disappears in the interior, the circumference of the embryo does not decrease; because the cells of the outer blastoderm spread out by division and flattening, and expand over the yolk-rich archenteron encompassing it, and thus compensate for the loss in terms of surface area.
By such active migration, cells of the animal hemisphere and of a belt of cells around the equator, termed the marginal zone, invaginate. The marginal cells passing over the lip pull along yolk-rich cells of the ventral blastoderm. All of these cells participate in forming the roof and floor of the archenteron. The archenteron becomes the principal cavity at the expense of the blastocoel.
Germ layer formation: The archenteron sometimes is termed mesendoderm because it gives rise to both the endoderm and mesoderm. The roof of the archenteron detaches from the floor; the roof comes to be the mesoderm (Fig. 5.7). We now arrive at the stage that displays the three conventional germ layers:
The endoderm: After the detachment of the roof of the archenteron, the walls of the remaining archenteron expand and converge to replace the lost roof. The archenteron has become the endoderm, it is identical with the primary gut where yolk released by the vegetal cells is dissolved.
The mesoderm (in early gastrulation also called the chordamesoderm) is the detached roof of the archenteron, an initially flat sheet of cells on the dorsal side lying between the closed archenteron and the outer blastoderm. Without delay, the median stripe forms the notochord, the adjoining lateral stripes form the metameric series of somites (Sect. 5.1.6).
The ectoderm: The outer wall of the gastrula is designated ectoderm; in the animal region of the gastrula it is also known as neuroectoderm, because the animal region will subsequently give rise to the nervous system.
Now the classical “germ layers” of a triploblastic animals are established.
5.1.5 Neurulation Prepares the Formation of the Central Nervous System
Neurulation is a process by which cellular material is sequestered to form the brain and spinal cord. The keyhole-shaped neural plate (Fig. 5.8) is formed above the detaching roof of the archenteron. The plate is delimited by neural folds that rise like surging waves and converge along the dorsal midline of the embryo. Here the folds adhere to each other and fuse, forming the hollow neural tube. An embryo undergoing these processes is called a neurula. While the tube is being formed, it sinks into the interior of the embryo and detaches from the surface. For some time closure of the neural tube is incomplete: an anterior and a posterior neuropore are left open.
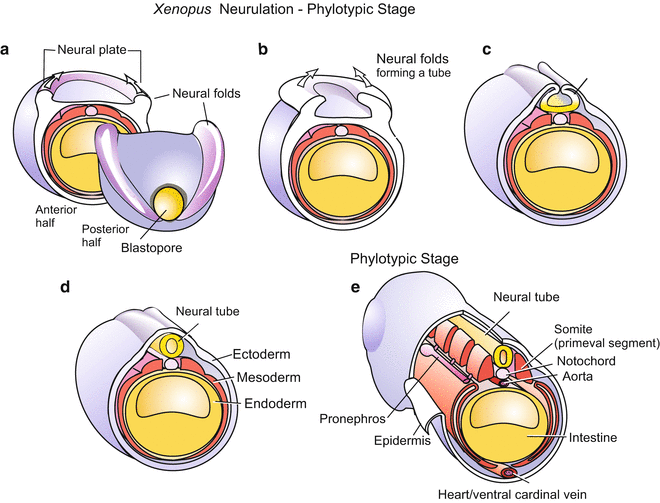
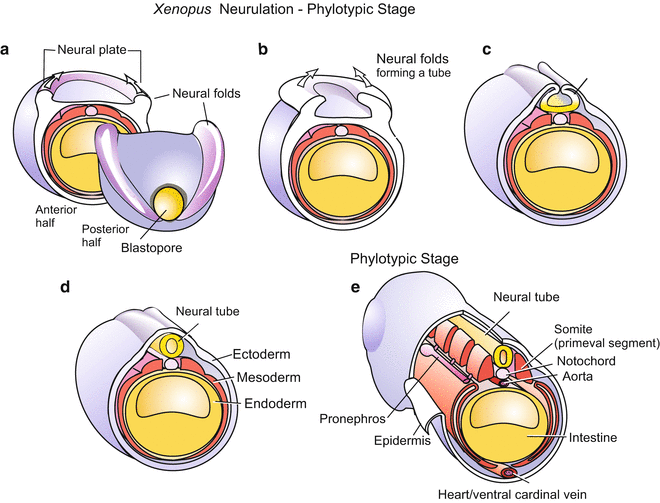
Fig. 5.8
(a–e) Neurulation in Xenopus. View onto the posterior half of the embryo. In (e) the phylotypic stage is reached
Above the neural tube, the ectoderm re-closes the outer wall. The anterior, expanding half of the neural tube will form the brain, while the posterior half will elongate to form the spinal cord. Residual cell groups along the neural tube, termed neural crest cells, will supplement the central nervous system by forming the spinal ganglia and the autonomic nervous system (Figs. 15.3 and 16.16). Further developmental potentials of the fascinating neural crest cells will be described in the Sects. 15.2 and 16.5.
5.1.6 The Mesoderm Gives Rise to Many Internal Organs: Notochord, Skeleton, Musculature, Heart, Blood, Kidneys and Some More
The detached chordamesoderm subdivides itself in a process of self-organization into several parts (Figs. 5.8, 5.9, and 5.10).
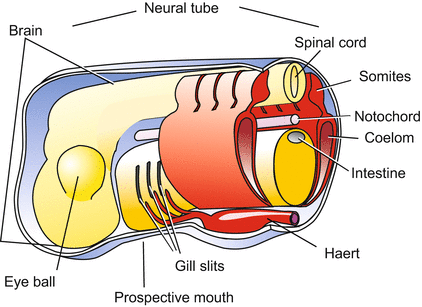
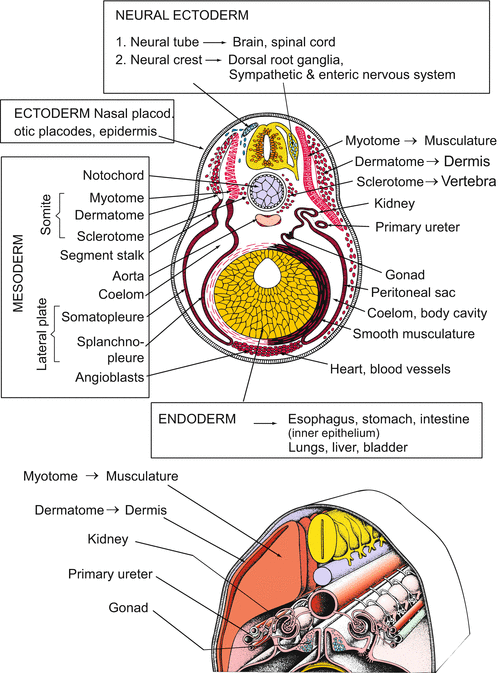
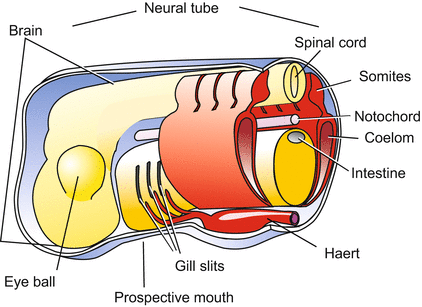
Fig. 5.9
Amphibians/vertebrates. Generalized basic body plan or phylotypic stage. By this stage, the basic traits of a chordate are elaborated: dorsal neural tube, notochord, foregut with gill slits, ventral heart (not shown). In addition, eye vesicles and somites are formed in all vertebrates
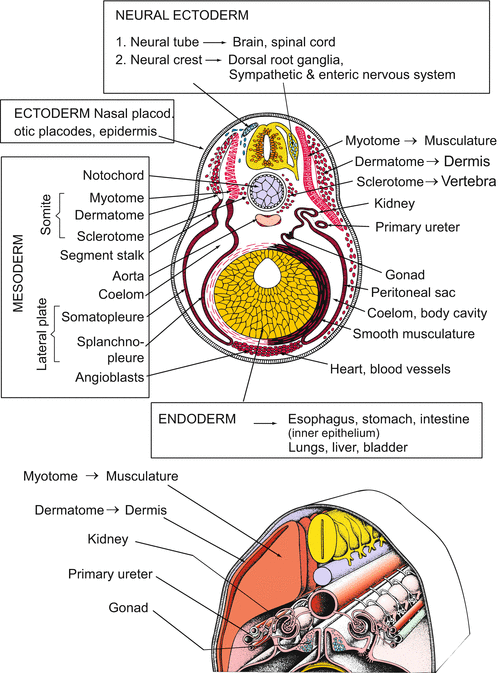
Fig. 5.10
Amphibian, generalized vertebrate. Development following neurulation. Fate of the ‘germ layers’ (after Portmann, top, and Huettner, bottom)
1.
The anterior part of the mesoderm which was the first mesoderm to involute, gives rise to the prechordal head mesoderm that forms the muscles of the head region.
2.
The head mesoderm is followed by the more posterior chordamesoderm. Along its midline, the round rod of the notochord detaches; the notochord is a transient structure needed to organize the development of the central nervous system CNS and the vertebral column.
3. and 4.
The dorsal axial mesoderm that is involved in notochord formation is flanked by two lateral bands, the paraxial or somitic mesoderm. These bands separate into block of cells called somites. Somites are also transient structures, but they are extremely important in organizing the basic segmental body pattern, and they give rise to many tissues: vertebrae, muscles, and the inner layer of the skin, called the dermis. Notochord and the two series of metameric somites are often subsumed under the term axial organs.
5. and 6.
The adjoining residual cells derived from the roof of the archenteron become flat, expanding sheets called lateral plates. Both plates spread laterally and ventrally into the space between the ectoderm and the endoderm. While doing so, a cleft within the plates transforms them into flattened bags. The bags enclose a new cavity, called the coelom or secondary body cavity. The coelom will subdivide into the pericardial cavity enclosing the heart, and the large body cavity. In mammals, this cavity is subdivided into the pleural cavity enclosing the lungs, and the peritoneal or visceral spaces around the intestine.
The wall of the lateral plate snuggling up and clinging to the ectoderm (somatopleure, or parietal mesoderm) will form the peritoneum and pleural lining, whereas the wall clinging to the endoderm (splanchnopleure) will provide the circular musculature of the stomach and the intestine, as well as the mesenteries needed to suspend the digestive tract in the coelom.
Somites and lateral plates are connected by intermediate mesoderm that forms a longitudinal series of thickenings, the pronephrotic rudiments. The solid rudiments hollow out and form funnels opening into the coelom. The pronephrotic rudiments will later be transformed and supplemented by further mesodermal derivatives to yield the urogenital system.
In the anterior body beneath the archenteron, where the two lateral plates approach each other, migratory mesodermal cells accumulate. They are myogenic and organize themselves to form the heart and the adjoining major blood vessels (Fig. 17.11).
The most extensive developmental potential belongs to the somites. Somites are transient but the cells constituting them do not vanish. They separate into two main groups: sclerotome and myodermatome. The myodermatome further subdivides into the myotome and dermatome (Fig. 5.10).
Sclerotome. The cells of the sclerotome lose contact with each other, emigrate, and accumulate at the notochord, thereby surrounding it. They become chondrocytes committed to construct the vertebral bodies. Most of the enclosed notochordal cells die, but some are left to form the nucleus of the intervertebral discs. These are the discs that slip in painful back injuries.
Myotomes. The packets of the myotomes expand and give rise to the cross-striated musculature of the body that occupies the largest spaces in the trunk and tail region.
Dermatome. The (dividing) cells derived from the dermatome migrate and spread extensively, clinging to the inner surface of the ectoderm. They will give rise to some additional muscle tissues but will mainly form the dermis, while the epidermis will be formed by the outer ectoderm covering the dermis.
The complete derivation of all connective tissue masses and skeletal elements is complex. Most precursors of the chondrocytes and osteocytes that construct the cartilage and bones of the body derive from the somites, but those osteocytes which form dermal bones appear to arise in the dermis. The skeletal elements in the ventral head and pharynx region, summed up as visceral skeleton, do not derive from mesoderm but from neural crest cells (Fig. 15.3).
5.1.7 From the Endoderm Arise: Gastrointestinal Tract, Lung, Liver, Pancreas and Bladder
The archenteron forms the pharynx with gills (of the tadpole) and lungs (of the adult), and the digestive tube with adhering organs such as pancreas, liver with gallbladder and the urinary bladder. The roof of the pharynx participates in forming the pituitary gland by contributing the anterior lobe, the adenohypophysis (Fig. 6.20). Yet, it is only the interior, epithelial lining of the gastrointestinal tract that derives from the endoderm; the musculature derives from the mesoderm (splanchnopleure), the nerve net from immigrated neural crest cells.
5.1.8 The Most Famous Embryological Experiments Were Done on Amphibians; Here: Cloning by Transplanted Nuclei, Identical Twins and Chimeras
Nuclear Transplantations.
Experiments that led to the production of cloned frogs are justly famous. Originally, the experiments were planned (by JB Gurdon (Nobel Prize 2012), R Briggs, TJ King, and others, see Box 1.1) not to clone animals but to examine whether somatic nuclei remain totipotent in the course of development, or if genetic information is irreversibly lost or inactivated (Fig. 13.2).
Identical Twins and Chimaeras.
One of the first experiments performed in developmental biology was carried out in 1888 by the German anatomist Wilhelm Roux. Under the magnifying glass were frog germs in the 2- (to 4-)cell stage. Using a hot needle, Roux killed one of the first two blastomeres. Because he did not remove the killed cell, he got crippled embryos with only one healthy half. Roux interpreted his observation as evidence that the fate each blastomere was already fixed and continued its development independently of other parts by “self differentiation”. His notion became known as “mosaic theory” of development. However, he also saw indications of some regulatory powers like those Hans Spemann could observe after him.
At about 1920 Hans Spemann re-examined Roux’s findings using a different technique. He separated early amphibian embryos (from the 2-cell stage up to beginning gastrulation) into halves using lassos made from baby hairs to make a ligature (Fig. 5.11). If the embryos were divided along the animal–vegetal axis so that each half was provided with material of the grey crescent, monozygotic (one-egg) identical twins arose. Partial constriction resulted in “Siamese” conjoined twins. By pressing together two cleaving embryos from the newts Triturus cristatus and Triturus vulgaris, chimaeras composed of a mosaic of genetically different cells were obtained.
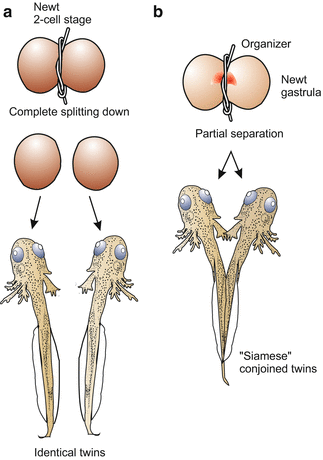
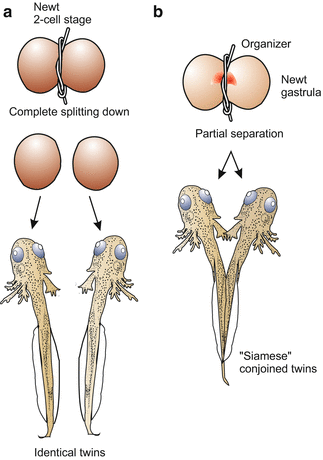
Fig. 5.11
Amphibian. Constrictions of newt embryos by a ligature performed by H. Spemann. Complete division results in monozygotic, identical twins, partial division leads to partial duplication of the body (“Siamese” twins in common parlance). Division of a blastula or early gastrula leads to a (entire or partial) duplication only when both halves get a piece of the Spemann organizer
Experiments with tissues from different origin, including tissues taken from different species, were continued in extensive transplantation studies (as follows).
5.1.9 By Means of Transplantations the Progress of Determination can be Analysed: Do Transplants Behave According to Their Location or Their Origin?
Among the most exciting experiments in the history of developmental biology were those carried out by Hans Spemann and his students between 1920 and 1940, using amphibians. The experiments yielded the first information on the significance of signals sent out by parts of the embryo to instruct other parts (embryonic induction). By microsurgery pieces were taken from donor embryos and inserted into host embryos at various places (Figs. 5.12, 5.13, and 5.14). The experiments were aimed at finding out whether tissue pieces would behave according to their (new) location, meaning that they still could be reprogrammed by positional cues, or whether they would behave according to their descent, meaning that they already were irreversibly committed.
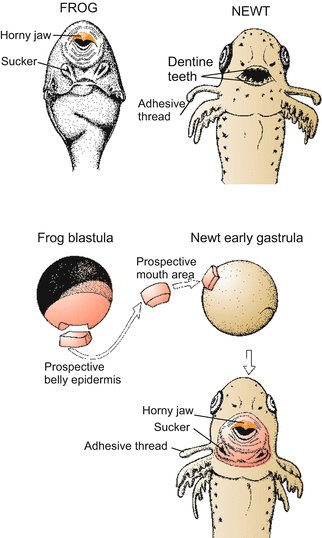
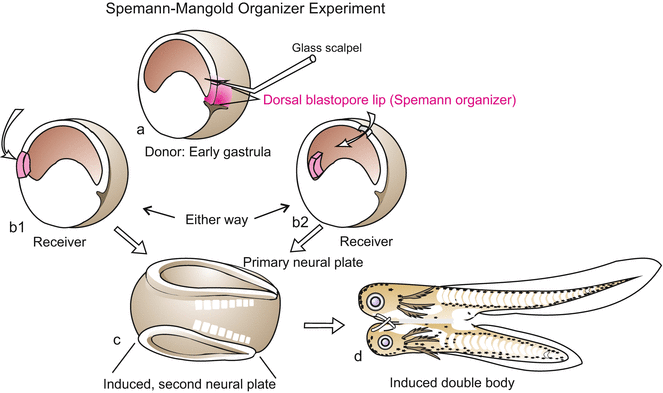
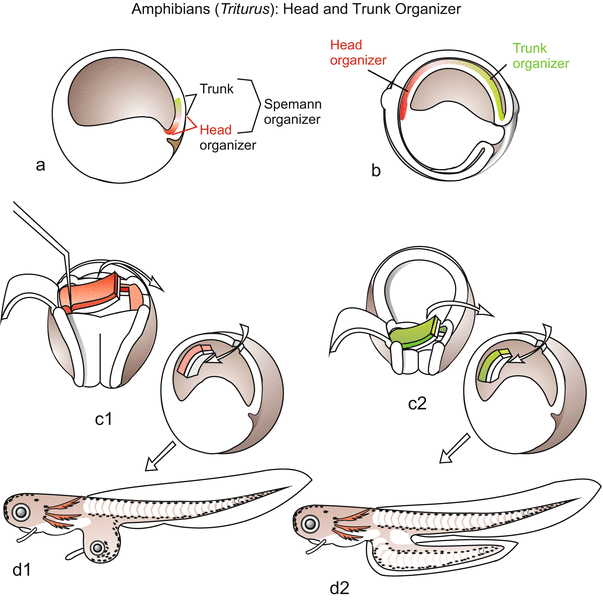
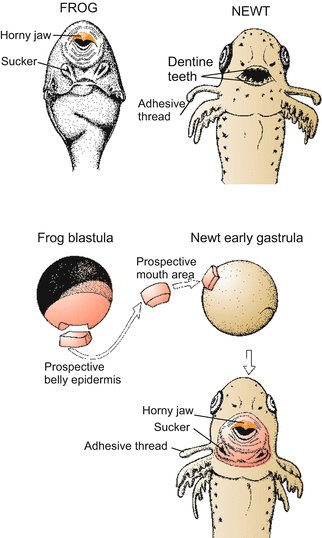
Fig. 5.12
Amphibian. Demonstration of positional information. Classic transplantation experiment of Spemann. A future (presumptive or prospective) piece of the epidermis of the belly, taken from a frog blastula, is transplanted into the future mouth region of a newt. The frog piece becomes committed in the mouth region of the host to form mouth tissue. However, it is a frog mouth that is formed, according to the genetic potential of the frog cells. Positional information is not species-specific, but the positional information received by a cell can only be interpreted according to the cell’s own genetic program
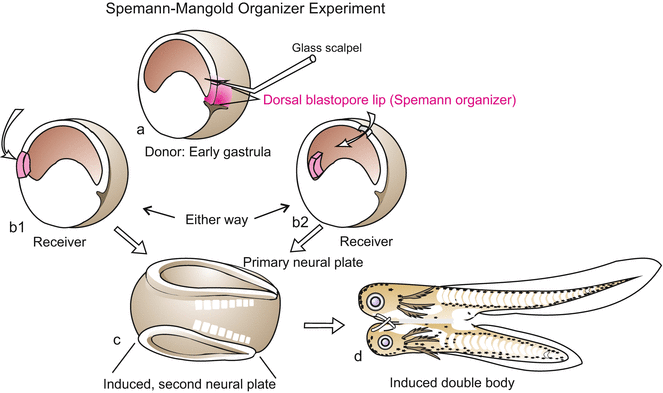
Fig. 5.13
Amphibian. Classic experimental induction of a secondary embryo by transplantation of an upper blastopore lip (‘organizer’). The transplanted tissue is marked in red, so it was shown that the secondary embryo is mainly formed by the host. Experiment proposed and interpreted by Hans Spemann and carried out by Hilde Mangold in 1924
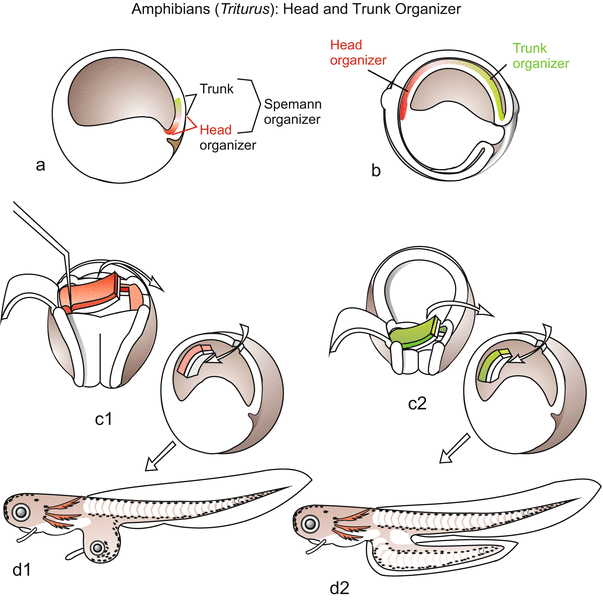
Fig. 5.14
Amphibian. Segregation of Spemann’s organizer into head organizer and trunk organizer. Upper part: After experiments by C. Niehrs 1998; lower part: Transplantation of parts of the roof of the archenteron, after O. Mangold 1933
In an experiment which addressed the question of positional information (Spemann: “development according to location”) the non-determined, prospective epidermis of the belly region was transplanted to the area fated to become the mouth region of a newt. The transplant formed mouth and teeth according to its new location (but horny teeth according to the genetic potency of the donor; tadpoles of frogs have horn teeth and not teeth of dentin as do salamanders! Fig. 5.12). In the course of such studies the phenomenon of induction was observed.
5.1.10 Systematic Transplantations Led to the Discovery of Embryonic Induction and of the Most Effective Signalling Centre, Now Known as the Spemann Organizer
Embryonic induction means: one part gives orders, the other responds. Systematically planned transplantation studies which began in 1918 lead to the discovery of extensive embryonic induction (published in 1924; for this discovery Spemann won a Nobel Prize for medicine in 1935, the second zoologist after Thomas Morgan to win it). In a classic example, the roof of the archenteron induces the overlying animal ectoderm to form the neural plate and, hence, the central nervous system (this is so-called “primary” or neural induction). But induction begins earlier and has a spectacular culmination when the early gastrula forms the blastopore. At this time and place the organizer becomes active.
Disregarding the actual sequence of inductive events in the early embryo for a moment, we tell the story of discovery in historical order. Prompted by Spemann, his student Hilde Mangold transplanted the upper blastopore lip of the darkly pigmented Triturus taeniatus into the ventral ectoderm or (accidentally) into the blastocoel of the white blastulae of Triturus cristatus (Fig. 5.13). The result was exciting: When properly inserted into the ventral ectoderm, the donor tissue did not adapt to the new surrounding. Instead, it started invaginating; soon axial organs (notochord, somites) and a head emerged, and eventually a complete second embryo formed, joined to the other: a Siamese twin possessing head, axial organs (notochord, neural tube), somites, etc. But even when the inserted lips dropped into the interior cavity, they initiated gastrulation in the surrounding host tissue. When the host embryo developed further, it was found that an additional system of axial organs appeared on the side where the graft came to lie. Partial or complete supernumerary embryos arose. Since black donor and white host embryos had been used, the supernumerary embryos were readily determined to be composed of host as well as donor tissue. In fact, the largest part of the supernumerary embryo was made by the host; host cells were induced to participate in formation of the second body.
Spemann referred to the upper blastopore lip as the organizer because it induces host cells to change their fate and is able to release an entire, coordinated developmental program leading to a well organized, complete embryo. A general term designating the source of an inducing signal is inductor or inducer.
It may be explicitly emphasized that organizer activity is not carried out by the same cell population all the time, because the cells that at this instant constitute the dorsal lip will in the next moment disappear into the interior and will be replaced by newly arriving cells. The term organizer designates a zone travellers pass through thereby being informed (induced) which path to take subsequently. In another paradigm the organizer is viewed as a classroom which students (cells) enter, and leave with new learned lessons.
A number of authors take the view that an intermediate signalling centre is inserted between the cortical rotation and the work of the Spemann organizer. First a preparatory emitter would be established in the area of the grey crescent (or its equivalent area in Xenopus), called Nieuwkoop centre. In the 32-cell blastula signals radiate from this centre committing the dorsally adjacent cells to act as the Spemann organizer. Other authors consider the Nieuwkoop centre and Spemann organizer to be two consecutive stages of the same signalling system. In both centres identical signalling molecules were detected, for instance WNT signalling molecules whose production is triggered by maternal β-catenin protein shifted into the posterior region of the egg during the cortical rotation. Read more about the important WNT-β-catenin (that often will be addressed in this book) in the following Sect. 5.1.12; a summary can be read in Chap. 11
5.1.11 The Organizer Separates into Head- and Trunk Organizer
Already in the young gastrula the Spemann organizer begins to display regional specificity. It splits into two to three initially overlapping fields that contribute differently to the induction of a second embryo.
A first field with inductive power is the region that encompasses the lip of the blastopore at the moment it is just being formed. The cells of this field are the first to flock together and to move into the blastocoel; they move anteriorly getting into the future head region and forming there the head mesoderm. This vanguard cohort of cells is responsible for the induction of the head with forebrain and midbrain.
The second field is initially located just above (in animal direction) the first field. Following the first cohort these cells invade the interior, too, and come to rest in the roof of the mid-archenteron; while they themselves are fated to become notochord they are responsible to induce the trunk.Stay updated, free articles. Join our Telegram channel
Full access? Get Clinical Tree
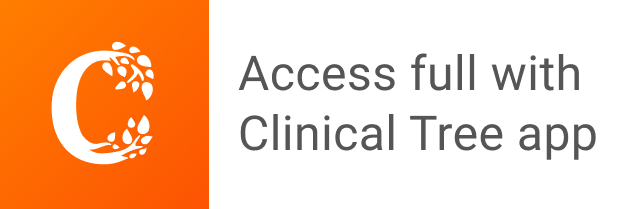