Chapter 14 Cyanobacterial Biointoxication in Free-Ranging Wildlife
The phylum Cyanobacteria (blue-green algae) is large and diverse, containing over 1000 species of oxyphototrophs.12 Prehistoric evidence suggests that photosynthetic cyanobacteria existed in shallow seas 3.8 billion years ago and were responsible for changing atmospheric water vapor, carbon dioxide, and nitrogen to the oxygen-dominated atmosphere of today. This evolutionary leap allowed development of higher multicellular life forms. Anaerobic bacteria, which had previously dominated the planet for over 1000 million years, were relegated to isolated areas devoid of oxygen.9 Because of their capacity for aerobic and anaerobic photosynthesis, cyanobacteria are able to thrive in a variety of habitats. In eutrophic surface water, they may multiply rapidly and form intense blooms.18 With global warming and increased eutrophication of certain water bodies, some cyanobacteria have significantly expanded their geographic ranges and have adapted to previously unsuitable environmental conditions.14
Cyanobacteria produce a large variety of biologically active organic compounds, many of which are toxic. These biotoxins are responsible for intermittent but frequently repeated intoxication events, affecting both wild and domestic animals. Toxins include microcystins, nodularin, anatoxins, saxitoxins, cylindrospermopsins, lyngbyatoxin a, and aplysiatoxins.2 They may be classified into five functional groups: hepatotoxins, neurotoxins, cytotoxins, dermatotoxins, and irritant toxins. These cell-based toxins may be released in high concentrations, with cell lysis.18 The most toxic freshwater cyanobacteria are Microcystis, Anabaena, and Panktothrix spp. (Table 14-1); the most commonly produced toxins are microcystins.8 The microcystins include seven cyclic aminopeptide hepatotoxins, with 65 structural isoforms currently described.3,7 The most common form is microcystin-LR, with the two variable l-amino acids being leucine (L) and arginine (R).9 Most microcystins have an LD50 of 60 to 70 µg/kg when administered intraperitoneally in mice.4 Microcystins cause hepatic cellular disruption, leading to sinusoid destruction and intrahepatic bleeding, with fatal hemorrhagic shock. Nodularin is a cyclic pentapeptide with a structure closely related to that of the microcystins and with similar hepatotoxic effects mediated through the potent inhibition of protein phosphatases. The alkaloid toxins are anatoxin, saxitoxins, cylindrospermopsin, and lyngbyatoxin a. Anatoxins function as depolarizing neuromuscular blocking agents in higher vertebrates. The toxins are not affected by acetylcholinesterase, resulting in excessive stimulation of muscle cells, followed by fatigue and paralysis. There is no known antidote and death is usually attributed to respiratory arrest. Saxitoxins are similarly neurotoxic; death is caused by muscle paralysis and respiratory arrest in mammals. Nerve cells are affected through inhibition of the opening of voltage-gated Na+ channels. Cylindrospermopsin is an irreversible inhibitor of protein biosynthesis, primarily affecting the liver, along with other major organs. Lyngbyatoxin a, a highly inflammatory toxin, is also a strong tumor promoter through activation of protein kinase C. Aplysiatoxins are commonly known for their dermatotoxic effects and cause inflammation of the skin. They are also potent tumor promoters.
TABLE 14-1 List of Known Cyanobacteria and Toxins Produced
Genus | Toxins Produced |
---|---|
Anabaena | Anatoxins, microcystins, saxitoxins |
Anabaenopsis | Microcystins |
Aphanizomenon | Saxitoxins, cylindrospermopsins |
Cylindrospermopsis | Cylindrospermopsins, saxitoxins |
Hapalosiphon | Microcystins |
Lyngbya | Aplysiatoxins, lyngbyatoxin a |
Microcystis | Microcystins |
Nodularia | Nodularin |
Nostoc | Microcystins |
Phormidium (Oscillatoria) | Anatoxin |
Planktothrix (Oscillatoria) | Anatoxins, aplysiatoxins, microcystins, saxitoxins |
Schizothrix | Aplysiatoxins |
Trichodesmium | Yet to be identified |
Umezakia | Cylindrospermopsin |
Adapted from Cyanosite: Cyanobacterial toxins, 2010 (http://www-cyanosite.bio.purdue.edu/cyanotox/toxiccyanos.html).
Factors Contributing to Growth and Toxicity of Cyanobacterial Blooms
The toxicity of a cyanobacteria bloom is influenced by a number of factors, including temperature, pH, light intensity, organic nutrients, and age of algal cells.8 Changes in toxin content occur on temporal and spatial scales. This probably reflects alterations in species and strain composition, with variations in toxins related to changes in environmental conditions influencing growth of the organism. At low winter temperatures, growth of Microcystis spp. may be negligible, but a sustainable viable population persists until temperatures start rising again. Growth of Microcystis aeruginosa is reported to be severely limited below 15° C, with optimal growth at 25° C to 32° C. However, maximal toxin production occurs at approximately 20° C. At temperatures of 32° C and 36°C, toxicity in cell culture was 1.6 and 4 times less than Microcystis spp. cultured at 28° C, suggesting that the level of toxicity is not correlated with greatest growth rate. Temperature changes are also reported to induce changes in peptide composition and concentration of toxins.11 The ability of cyanobacteria to grow at higher temperatures gives them a competitive advantage over other phytoplankton species, such as diatoms and green algae. Warming of surface waters also increases vertical stratification of water bodies, reducing vertical mixing. With increased global temperature trends, this is occurring earlier in spring and persisting until later in autumn, which increases the growth period for cyanobacteria.14 At favorable light intensities, M. aeruginosa will undergo an initial lag phase of 5 days, followed by an exponential growth phase of approximately 11 days. As light intensity increases, growth rate declines. Gas vacuole content and buoyancy of the cells are also influenced by light intensity, initially increasing as the brightness increases and then decreasing. Buoyancy is regulated by a complex relationship between light and composition changes in protein-to-carbohydrate ratios within the cyanobacterial cells. This characteristic is important in the pathogenesis of toxicosis because most animals drink from the surface of water bodies. Blooms of Microcystis spp. and increased microcystin concentrations have been associated with eutrophication, characterized by higher water nitrogen-to-phosphorus ratios. These are often caused by addition of nutrients derived from human wastes from mining, industrial, and forestry activities, as well as sewerage, or the input of fertilizers or animal wastes from intensive farming. Availability of Zn2+ and Fe2+ is also reported to influence to toxin yields because Fe2+ is required for nitrogen assimilation, respiration, and chlorophyll synthesis.
Toxicity in Animal Species
Blooms caused by various cyanobacteria species have been associated with mortalities in fish, turtle, birds, and mammals worldwide. Bivalves are able to ingest cyanobacteria toxins by filtering water containing cyanobacteria for their food. Contradictory reports have suggested that they may promote toxic blooms through passing live Microcystis spp. in their pseudofeces or reduce blooms by ingesting the Microcystis spp., clearing the water of the organism. Several bivalves have been shown to be able to accumulate and store toxins, with relative resistance to the toxin themselves. Crayfish tolerate and grow well on Microcystis spp. Toxins accumulate in the intestines and hepatopancreas, with no apparent harmful effects. Because of the bioaccumulation of cyanotoxins, including microcystin, nodularin, and saxitoxin in these invertebrates, they are potential sources of these toxins for the animals and humans that consume them.18 It should be stated however, that evidence that supports the transfer of microcystins within the food web and biomagnification of the toxin is currently limited. Variations occur in these two processes depending on the toxin involved, and efforts to determine the effects of cyanotoxins in aquatic food webs are frequently complicated by a multitude of stress factors that occur concurrently during blooms.4
Mass mortalities of fish have been linked to the secretion of microcystins in association with algal blooms. Potential contributing factors include low water level, high water temperature, high pH, high ammonium concentration, low dissolved oxygen concentration, and the presence of other toxin-producing microorganisms.4,7 Fish are exposed to toxins during feeding or when toxins pass over their gills during breathing. In early life stages of fish, microcystins interfere with developmental processes and organ function. These include alterations in time to hatching of embryos and dose-dependent decreases in survival and growth rates of juveniles. The most pronounced effects are poor yolk resorption, small head, curved body and tail, hepatobiliary abnormalities, alterations to hepatocytes, and cardiac problems. Chronic toxic effects are hypothesized to be caused by increased energy demands required by the detoxification systems in these life stages.
In adult fish, the main organs affected by microcystins are the kidneys and liver with pathology similar to that described for mammals, including a loss of liver structure resulting in necrosis and loss of function.18 The toxin binds protein phosphatases 1 and 2A, which causes an increase in reactive oxygen species in hepatocytes, leading to oxidant shock and cell necrosis.7 Nodularin is reported to have similar hepatotoxic effects in fish. Epithelial cells of the gills undergo cellular degeneration and necrosis. Gill cell chloride ion pumps may be disrupted affecting osmotic balance. Cardiac effects from environmentally relevant microcystin concentrations include increased heart rate, stroke volume, and cardiac output. Variations in sensitivity to the toxin have been demonstrated among different species of fish. This may be related to selective feeding of toxic or nontoxic strains of Microcystis, duration of digestion, and the number of lysed cells releasing toxins. Species native to eutrophic habitats are generally more resistant than those found in oligotrophic habitats. It has been demonstrated that cyanobacterial hepatotoxins may accumulate in fish tissue, especially the liver and, to a lesser extent, in muscle and viscera. These may be transferred up the food web, constituting a risk to piscivorous birds, animals, and humans that consume these fish.
In recent years, there have been reports of mass mortalities of lesser flamingos in East Africa—30,000 birds at Lake Bogoria, Kenya in 2000 and at Lake Embagai, Tanzania, 800 birds died during 2003. During 2004, deaths of large numbers of birds occurred in two alkaline lakes, Big Momela and Manyara, Tanzania, with 15,000 reported fatalities at Lake Manyara during a 2-month period. The cause of these deaths is presumed to be the consumption of the cyanobacterium Arthrospira fusiformis. This is an important natural food item for these birds and, until recently, was considered a nontoxic species. A. fusiformis has recently been shown to produce microcystins and anatoxin a.6 Birds intoxicated with anatoxin a develop symptoms of staggering, muscle fasciculation, hyperpnea, cyanosis, paralysis, hyperesthesia, opisthotonus, convulsions, and death caused by respiratory failure.3,18 A number of theories have been proposed for the pathogenesis relating to these flamingo deaths, including the existence of toxic and nontoxic strains of A. fusiformis, changes in environmental conditions that allow this strain to become toxic, toxin level variations through the lifespan of the organism, and cellular concentration in the water column.
In South Africa, cyanobacterial blooms are an increasing problem in freshwater bodies. This is predominantly caused by progressive eutrophication but has been influenced by the general trend of increasing environmental temperatures in the Southern Hemisphere since the 1950s.13
Cyanobacterial blooms on a water body are frequently variable in a spatial context, with patches of high and low concentrations of the algal elements. Winds push the floating cyanobacteria cells so that they accumulate on the leeward shore of water bodies and tend to leave the windward shore free of cyanobacteria.16 Gas vacuoles of hollow proteinaceous vesicles filled with air facilitate buoyancy regulation in cyanobacteria, allowing them to access near-surface light energy and the more nutrient-rich deeper waters.6 It has been observed that cattle, sheep, goats, and wildlife will consume this concentrated scum of M. aeruginosa, although they have the option of avoiding these denser portions of the bloom. Laboratory mice will preferentially consume dense cultures of this harmful cyanobacteria rather than limpid water. The reasons for this preferential selection have not been determined, but the mice did not appear to differentiate between toxic and nontoxic strains of the cyanobacteria. The mice reportedly had to consume up to 90% of the lethal dose before any measurable adverse effects were manifested.
Clinical Signs and Treatment
Susceptibility to cyanobacteria toxins varies in domestic species. Anatoxins most often affect pigs and dogs, with waterfowl susceptible and ruminants relatively resistant. The quantity of toxin consumed influences onset and duration of clinical symptoms, from peracute to chronic. In peracute cases, hepatotoxins result in death within hours, frequently without any premonitory signs. Acutely to subacutely affected animals have symptoms associated with liver damage, including icterus and hepatogenic photosensitization. Other symptoms include vomiting, lethargy, ruminal stasis, constipation, and diarrhea. Death usually occurs in 1 to 2 weeks. Chronic cases usually result from the ingestion of sublethal doses over a prolonged period and typical symptoms are emaciation, inappetence, and chronic photosensitivity, with alopecia. In severe cases, hepatic enzyme levels may be elevated and coagulation parameters altered, with hyperbilirubinemia and hypoglycemia present. Treatment of hepatotoxicity is symptomatic and supportive,3 although implementation in free-ranging wildlife may be challenging. Many species do not adapt well to captivity or being restrained to allow administration of treatment regimens.
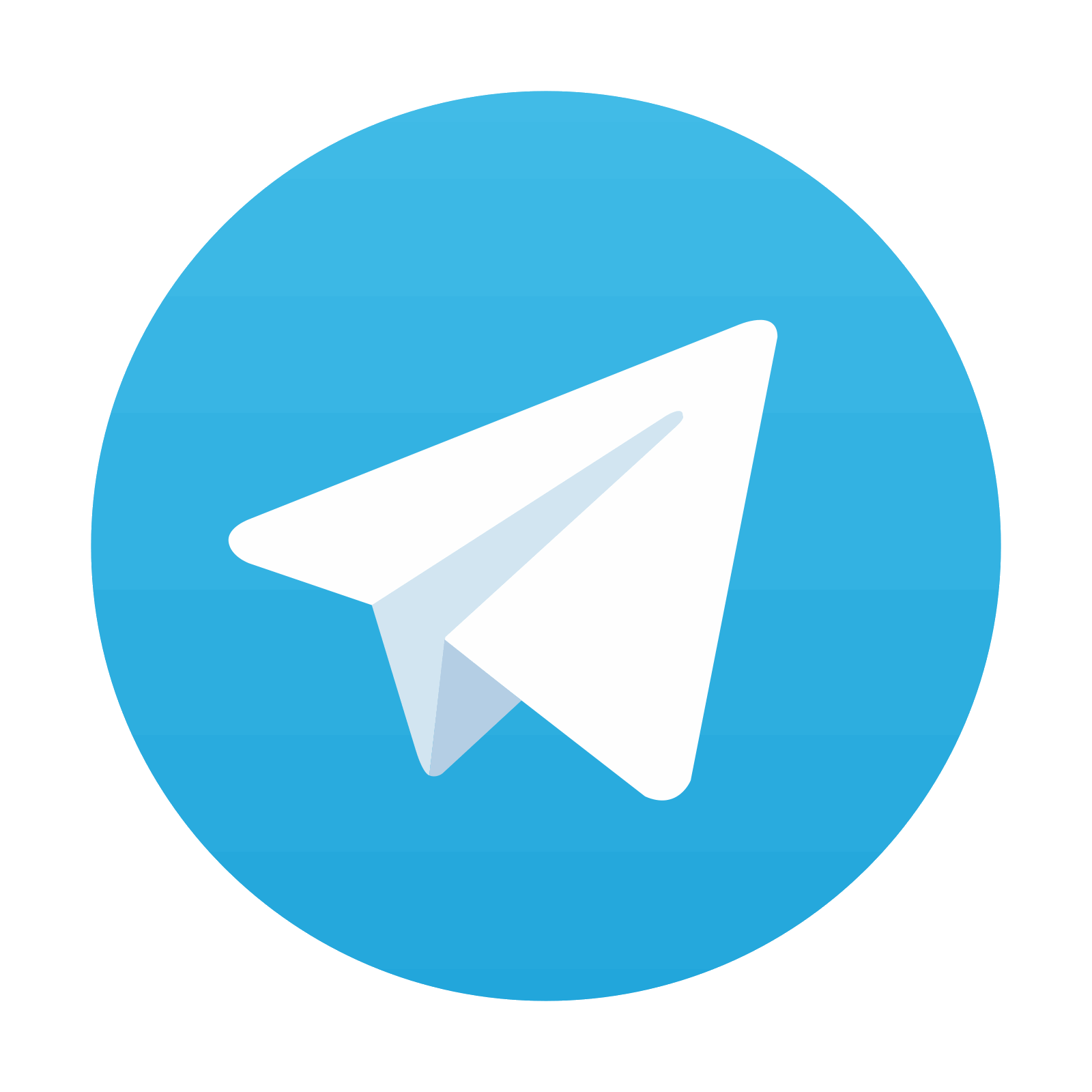
Stay updated, free articles. Join our Telegram channel
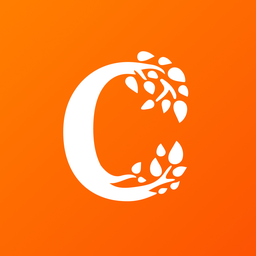
Full access? Get Clinical Tree
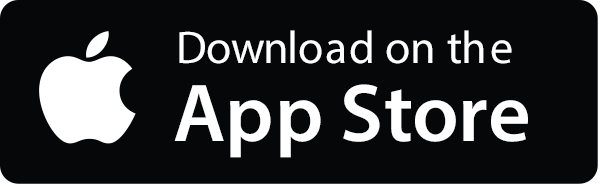
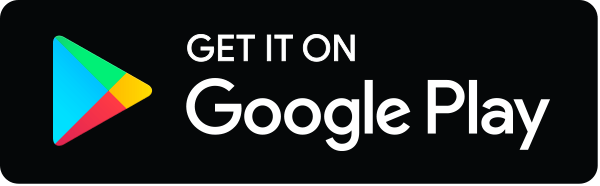