Allison C. Leonard Celine Stoica and Georgina Cox The misuse and overuse of antimicrobials has promoted the evolution and spread of antimicrobial resistance (AMR), which threatens our ability to effectively treat infections (Van Boeckel et al. 2015; Brannon and Hadjifrangiskou 2016). The AMR crisis is one of the most significant threats to human health today (World Health Organization 2015; Council of Canadian Academies 2019) and a contributing factor has been the extensive use of antimicrobials in the animal industry. Indeed, antimicrobials have been used for more than 70 years as growth promoters to improve the feed‐to‐weight ratio (Van Boeckel et al. 2015; Stoica and Cox 2021). In terms of mass consumption, antimicrobial use in food animal production far exceeds that in human consumption (Van Boeckel et al. 2015). If these trends continue, consumption is projected to increase 67% by 2030 (Van Boeckel et al. 2015). Although antimicrobial stewardship practices are increasing and regulations are being introduced to monitor antimicrobial consumption in humans, the same measures have been more limited in the animal industry (Van Boeckel et al. 2015). Numerous countries are taking steps toward changing their animal husbandry practices, including restricting the use of antimicrobials that are important for human medicine (Stoica and Cox 2021). Although AMR transmission rates have decreased with the introduction of the abovementioned restrictions, banning the use of antimicrobials in the agricultural sector is not a viable option and it is imperative that novel One Health‐centered approaches are investigated to take action in controlling and treating infectious diseases in livestock as well as in humans (Stoica and Cox 2021). Traditional antimicrobial chemotherapeutic approaches work by either inhibiting growth or killing the bacteria, but this inadvertently enables resistant isolates to proliferate (Rasko and Sperandio 2010; Allen et al. 2014). An alternative approach to development of antimicrobial agents, which could circumvent the resistance limitations associated with traditional antibiotics, is targeting the microbial virulence factors that determine pathogenicity. Bacterial pathogens harness an impressive array of virulence factors to colonize, infect, and ultimately damage the host (Allen et al. 2014; Dickey et al. 2017; Stoica and Cox 2021). Virulence factors enable processes such as adhesion, biofilm formation, motility, toxin secretion, immune evasion, colonization, and bacterial cell–cell communication (i.e. quorum sensing, QS) (Figure 6.1; Chapter 1; Rasko and Sperandio 2010; Theuretzbacher and Piddock 2019). Antivirulence strategies are designed to target and neutralize these mechanisms, ultimately inhibiting pathogenicity (Allen et al. 2014; Stoica and Cox 2021). Since these approaches are focused on disarming the pathogen, rather than killing or inhibiting growth, it is assumed they will be less susceptible to the development of resistance (Rasko and Sperandio 2010; Allen et al. 2014; Theuretzbacher and Piddock 2019; Stoica and Cox 2021). Although resistance to antivirulence agents has been described (Allen et al. 2014), it is unlikely such mechanisms will disseminate in the environment due to the reduced selective pressure of these approaches (Stoica and Cox 2021). Importantly, antivirulence agents are often pathogen‐specific precision therapeutics lacking the broad‐spectrum activity associated with numerous antibiotics (Spaulding et al. 2018). The relatively wide range of anti‐tuberculosis agents targeted to specific mycobacterial targets illustrates this point well. As such, an additional advantage is that they would be less likely to disrupt the microbiota (Stoica and Cox 2021). Furthermore, the cost of developing antibacterial agents will be far lower for narrow‐spectrum agents targeted to important animal and human diseases than the development of broad‐spectrum agents. In this chapter, we highlight attractive pathogenesis‐based targets for the development of next‐generation agents to combat bacterial infections, which could diminish the severity of the AMR crisis. Because of space limitations, in some instances reviews are referenced in place of primary research articles. Bacterial adhesion to host cells and tissues is often a critical and early component of colonization and infection. By adhering, bacteria resist physical clearance by host cleansing mechanisms (Leonard et al. 2019). In some instances, adhesion also facilitates toxin secretion, cell invasion and nutrient acquisition (Leonard et al. 2019). Furthermore, many bacterial pathogens subsequently form biofilms, which can further enhance virulence (Stoica and Cox 2021). Bacteria initially interact via weak and transient interactions, which are strengthened by specific and high‐affinity interactions between bacterial adhesins and host ligands (Mühlen and Dersch 2016; Leonard et al. 2019). Pathogenic bacteria use an impressive arsenal of surface appendages to facilitate this process, including long hair‐like organelles such as pili/fimbria, non‐polymeric proteinaceous adhesins, glycopolymers and complex multimeric proteinaceous assemblies (Mühlen and Dersch 2016; Leonard et al. 2019). Targeting these host–pathogen interactions through the development of anti‐adhesive strategies could reduce colonization and infection rates (Leonard et al. 2019). There are numerous potentially efficacious approaches, including interference with adhesin or host receptor biosynthesis/surface presentation and physically blocking the interaction of adhesins with host receptors (Figure 6.2a; Brannon and Hadjifrangiskou 2016; Leonard et al. 2019). Bacterial adhesins often have a strong affinity for specific host receptors, which contributes to host tissue tropism; this is one reason that some bacteria have a narrow host range (Pickering et al. 2019). An example is the opportunistic canine pathogen Staphylococcus pseudintermedius, one of the leading causes of skin infections in dogs (Chapter 25), which possesses the canine‐specific fibrinogen adhesin SdsL (Pickering et al. 2019). As such, anti‐adhesives could potentially be developed for each clinical scenario and the desired host (Spaulding et al. 2018). Staphylococcus aureus is a major human and animal pathogen (Chapter 25; Sakwinska et al. 2011). Strains of S. aureus produce up to 25 cell wall‐anchored (CWA) surface proteins (Leonard et al. 2019), involved in host cell adhesion, biofilm formation, immune evasion, inflammation, iron acquisition and host cell invasion (Foster et al. 2014). The housekeeping transpeptidase, sortase A (SrtA), covalently anchors the majority of these CWA proteins to the peptidoglycan (Mazmanian et al. 2000). Importantly, SrtA deletion does not impact bacterial growth and mutants exhibit significantly reduced virulence (Mazmanian et al. 2000). As a result, SrtA has been the target of numerous drug discovery efforts, resulting in the identification of inhibitors with in vivo efficacy (Leonard et al. 2019). The most promising examples are 3,6‐disubstituted triazolothiadiazoles that inhibit SrtA activity without exhibiting anti‐S. aureus activity or host cell cytotoxicity (Leonard et al. 2019). Figure 6.1 Common bacterial virulence factors. Bacteria harness an array of virulence factors to colonize and infect the host. Surface‐associated adhesive proteins, flagella and pili are often required for host cell adhesion, colonization and biofilm formation. Pili and flagella also enable bacterial motility. Quorum sensing (QS) and two‐component systems are often involved in the regulation of virulence factor gene expression. Surface‐associated proteins can also promote host cell invasion and immune evasion, as well as the acquisition of essential nutrients (e.g. iron) from the host environment. Specialized secretion systems transfer virulence factors into the external environment and/or into host cells. Secretion systems can also facilitate the transfer and uptake of antimicrobial resistance‐associated genetic material. Efflux pumps extrude antibiotics, host‐derived toxic molecules and virulence factors (e.g. siderophores), which contributes to bacterial survival in the host. Certain virulence factors, such as lipopolysaccharides (LPS), are strong stimulators of the host’s immune response. See also Figures 1.2 and 4.1. Figure 6.2 Overview of proposed pathogenesis‐based strategies to combat bacterial infections. (a) Anti‐adhesives reduce bacterial host surface attachment and colonization. Inhibitors can target the host receptor–adhesin interaction or inhibit receptor/adhesin biosynthesis. (b) Biofilm inhibitors degrade extracellular polymeric substances (EPS) to prevent/disrupt biofilm formation. Biofilm inhibitors could increase bacterial susceptibility to antibiotics and enhance clearance by the host’s immune system. (c) Quorum sensing (QS) and two‐component systems (TCS) regulate numerous virulence‐associated genes. QS inhibitors (top), also known as quorum quenchers, neutralize/degrade autoinducers or competitively bind to QS receptors, both of which prevent QS signaling, thus decreasing virulence gene expression. TCS inhibitors (bottom) target the sensory domain of the histidine kinase or prevent activation of the response regulator. (d) Antimotility agents (e.g. flagella or pili inhibitors) inhibit bacterial swimming and/or swarming, often attenuating virulence. These inhibitors can also impact flagella‐ or pili‐mediated adhesion. (e) Targeting toxin‐mediated damage involves neutralizing the toxin directly (e.g. antibodies or peptides) (left) or inhibiting the toxin’s secretion system (right). Inhibiting secretion systems could also prevent the secretion of numerous virulence factors (e.g. adhesins, proteases, lipases, curli fibers, and other virulence‐associated factors) and the transfer of antimicrobial resistance‐associated genetic material. (f) Phage therapy (left) uses engineered phage DNA to integrate into virulence genes, reducing expression. In addition, phage‐encoded virulence gene repressors, phage‐derived molecules, QS modulators or virulence factor/toxin neutralizers, could also attenuate virulence. Efflux pump inhibitors (right) could reduce the extrusion of virulence factors (e.g. siderophores), antibiotics and inhibitory host‐derived molecules (e.g. bile salts), thereby reducing efflux‐associated virulence, increasing antibiotic susceptibility and decreasing survival in the host environment. Although the repertoire of CWA proteins can vary between strains, several are highly conserved. Targeting the production and surface presentation of these adhesins (e.g. through SrtA inhibition), or blocking specific adhesin‐host ligand interactions, could form the premise of novel anti‐staphylococcal therapies. For example, adhesion to the host ligand fibronectin, which is mediated by the CWA fibronectin‐binding proteins (FnBPs) A and B, facilitates S. aureus host‐cell invasion (Petrie et al. 2020). Through the invasion and internalization of host cells, S. aureus becomes sheltered from the inhibitory effects of antibiotics and the host’s immune response, promoting chronic and persistent infections (Shkreta et al. 2004; Petrie et al. 2020). Anti‐adhesives targeting fibronectin adhesion could reduce the occurrence of host cell invasion and the instances of chronic infections (Petrie et al. 2020). Another promising target is clumping factor B (ClfB), the major keratin/loricrin adhesin involved in skin and nasal colonization (Leonard et al. 2019). Since ClfB is important for persistent colonization, inhibitors targeting the ClfB‐loricrin/keratin protein–protein interaction could potentially be used for decolonization efforts (Leonard et al. 2019). Finally, clumping factor A (ClfA)‐mediated fibrinogen adhesion also significantly contributes to virulence, enabling colonization of indwelling medical devices, cell–cell clumping and immune evasion (Petrie et al. 2020). In the dairy industry, S. aureus is one of the leading causes of bovine mastitis, accounting for up to 75 million infections globally (Sakwinska et al. 2011). Both ClfA and FnBPs have been associated with bovine mastitis (Shkreta et al. 2004); anti‐adhesives targeting these proteins could reduce the burden of bovine mastitis. In addition to afimbrial adhesins, bacteria may use pili – or fimbriae – to adhere to the host (Mühlen and Dersch 2016). These hair‐like structures composed of multiple immunoglobulin‐like subunits (Mühlen and Dersch 2016) play important roles as virulence factors in many burdensome human and animal pathogens (Mühlen and Dersch 2016). For this reason, pilicides targeting biosynthesis have been investigated extensively. Pili biogenesis uses the chaperone usher assembly pathway, which relies on periplasmic chaperone proteins that stabilize and transport pilus subunits to the usher in the outer membrane for assembly (Pinkner et al. 2006; Mühlen and Dersch 2016). “Pilicides” targeting uropathogenic Escherichia coli have shown promise in vitro, inhibiting hemagglutination and biofilm formation, and ex vivo, inhibiting host‐cell adhesion (Pinkner et al. 2006). Pilicides include bicyclic 2‐pyridones that prevent essential chaperone proteins from interacting with the various pili subunits (Pinkner et al. 2006). Pilicides, which importantly do not inhibit growth, might show efficacy against a range of pathogens because of conservation of the pili biogenesis pathway (Mühlen and Dersch 2016). Typically, pili adhere to surface receptors through a specific interaction with terminal pili subunits and host receptor sugars or peptides (Mühlen and Dersch 2016). This interaction can be inhibited; for example, mannosides have been described that function as glycomimetics of the mannosylated type 1 pili host receptor, which competitively inhibit adhesion of the FimH pili tip adhesin to the urinary tract and the intestines (Spaulding et al. 2018). In addition, mucin glycoproteins have similar glycosylation patterns to host‐cell receptors and inhibit bacterial adhesion to highly glycosylated receptors (Parker et al. 2010; Mühlen and Dersch 2016). Muc1, a mucin derived from cows’ milk, reduces adhesion of bacteria such as E. coli, Pseudomonas aeruginosa and Helicobacter pylori to human intestinal cells (Parker et al. 2010). Furthermore, synthetic adhesin‐mimicking peptides, or antibodies that recognize an adhesin host‐binding epitope, can also be used to inhibit adhesion (Mühlen and Dersch 2016). Finally, adhesion can also be inhibited from the host perspective using anti‐adhesives targeting receptor biogenesis and surface presentation (Mühlen and Dersch 2016); glycosphingolipids are common receptors for mucosal pathogens and biosynthesis inhibitors (e.g. N‐butyldeoxynojirimycin) reduced adherence of P‐fimbriated E. coli in the murine urinary tract (Svensson et al. 2003). Following surface adhesion, many bacterial pathogens subsequently form biofilms, which is a process largely controlled by QS mechanisms. Biofilms consist of surface‐associated bacterial communities enclosed in extracellular polymeric substances (EPS), which form a matrix of high molecular weight polymers, such as polysaccharides, DNA and proteins (Fleming and Rumbaugh 2018). Importantly, infections associated with biofilms are particularly difficult to treat (Mühlen and Dersch 2016). Biofilms are associated with livestock pneumonia, liver abscesses, various forms of enteritis, mastitis, and wound infections (Clutterbuck et al. 2007). The protective EPS matrix, in combination with the close proximity of the bacterial cells, also allows for the rapid transfer of AMR‐associated genetic material, as well as decreased susceptibility to antibiotics, the host’s immune defenses and other environmental stressors (Mühlen and Dersch 2016; Stoica and Cox 2021). A wide array of anti‐biofilm agents have been described (Rajput et al. 2018; Stoica and Cox 2021), which include inhibitors of initial surface attachment, the QS systems responsible for biofilm associated gene expression, production of the EPS matrix and matrix degradation (Cegelski et al. 2008). The development of agents that initiate cellular dispersal and eradication of established biofilms could be particularly impactful; for example, this could be achieved through the degradation and/or disruption of the DNA, protein, or polysaccharides of the EPS matrix (Figure 6.2b; Fleitas Martínez et al. 2019). Indeed, cell dispersal is a natural stage of the biofilm life cycle, enabling relocation to a new colonization site; examples of biofilm dispersing molecules and enzymes already exist in nature and could be harnessed for such an approach (Mühlen and Dersch 2016). For instance, DNase I degrades DNA in the EPS matrix, disrupting and destabilizing biofilms in Bordetella pertussis, Listeria monocytogenes, and Campylobacter jejuni (Mühlen and Dersch 2016; Fleitas Martínez et al. 2019). Dispersin B, produced by Actinobacillus actinomycetemcomitans, hydrolyzes glycosides and disrupts established Actinobacillus biofilms and also inhibits the formation of S. aureus biofilms (Kaplan et al. 2004; Izano et al. 2008). In addition, the combination of biofilm dispersing agents with antibiotics can enhance antibiotic efficacy (Mühlen and Dersch 2016). It is important to acknowledge that biofilm dispersal treatments could lead to worse outcomes, such as bacteremia, if not used in conjunction with antimicrobial agents (Fleming and Rumbaugh 2018). While eradicating biofilms is challenging due to the protective nature of the EPS matrix and close proximity of cells, agents that prevent surface adhesion – or EPS formation – could prevent biofilm establishment (Figure 6.2b; Fleitas Martínez et al. 2019). The QS inhibitor coumarin reduced biofilm formation, swarming motility and fimbrial adhesion in E. coli O157:H7 without impacting growth (Fleitas Martínez et al. 2019). As described below, biofilm formation is controlled by QS mechanisms and many QS inhibitors also inhibit biofilm formation (Stoica and Cox 2021). Bacteria use QS systems to communicate and coordinate responses important for colonization and infection (Rutherford and Bassler 2012). Chemical signaling molecules, or autoinducers, are secreted and monitored by a bacterial population. When they surpass a certain threshold that is correlated with cell density, the autoinducers bind to cellular receptors, triggering a signaling cascade that alters the expression of a wide array of genes (Rutherford and Bassler 2012). Importantly, QS controls many virulence mechanisms, such as motility, biofilm formation and the production/secretion of degradative enzymes and toxins (Stoica and Cox 2021). Owing to the importance of QS in bacterial virulence, numerous inhibitors of natural and synthetic origin, often termed “quorum quenchers,” have been described. In particular, P. aeruginosa is a readily used model for quorum quenching drug discovery efforts (Theuretzbacher and Piddock 2019). While QS systems vary among bacteria, there are common interspecies signaling molecules (e.g. Autoinducer‐2) that could represent more broad‐spectrum targets (Stoica and Cox 2021). Overall, there are a number of ways to interfere with QS, including inhibition of autoinducer biosynthesis, inactivation of the autoinducer and interfering with QS signal receptors (Figure 6.2c; Fleitas Martínez et al. 2019). Autoinducer biosynthesis inhibitors are often substrate or transition state analogs of biosynthetic enzymes (Fleitas Martínez et al. 2019). For example, transition state analogs can inhibit QS systems in enterohemorrhagic E. coli O157:H7 and Vibrio cholerae (Gutierrez et al. 2009). In P. aeruginosa, anthranilic acid analogs disrupted the MvfR‐dependent QS system, reducing virulence in a murine model of systemic infection (Lesic et al. 2007). Furthermore, in Streptococcus mutans, mucin O‐glycans suppress QS mechanisms, preventing biofilm adherence (Werlang et al. 2021). These mucins are also effective at inhibiting DNA transformation, reducing the rate of AMR gene transfer (Werlang et al. 2021). More broad‐spectrum inhibitors have also been described for Gram‐positive pathogens. For example, ambuic acid inhibits QS signal biosynthesis in several Gram‐positive pathogens, including the agr QS system in S. aureus (Todd et al. 2017). The enzymatic inactivation of extracellular autoinducers occurs naturally in microbial populations (Fleitas Martínez et al. 2019). Well‐characterized examples include lactonases, acylases, and oxidoreductases, which degrade acylated homoserine lactone QS molecules (LaSarre and Federle 2013). Numerous quorum quenching enzymes have shown efficacy in animal models of infection (Fleitas Martínez et al. 2019). Finally, inhibitors of QS signal detection, via competitive and noncompetitive inhibitors of autoinducer receptors, have also been described (Fleitas Martínez et al. 2019). For example, a P. aeruginosa QS analog inhibitor, meta‐bromo‐thiolactone, inhibits the LasR and RhlR receptors, attenuating virulence and biofilm formation (O’Loughlin et al. 2013). In addition, flavonoids allosterically inhibit these receptors (Paczkowski et al. 2017). Under certain circumstances, it may be beneficial for antivirulence agents to be used in conjunction with antibiotics to increase efficacy (Stoica and Cox 2021). For example, furanone C‐30 is a synthetic anti‐QS compound that competitively binds the LasR QS receptor in P. aeruginosa (Stoica and Cox 2021). When used in conjunction with various antibiotics, furanone C‐30 demonstrated synergistic effects against antibiotic resistant strains of P. aeruginosa (Stoica and Cox 2021). In summary, quorum quenching is an attractive antivirulence approach with numerous described inhibitors. However, in some instances the inhibition of QS has been reported to promote other pathogenic processes, which is a limitation that needs to be considered when developing such agents (Stoica and Cox 2021). Prototypical two‐component systems (TCSs) consist of a sensor kinase that responds to environmental signals and modifies a cognate response regulator that then performs a biochemical function such as modulation of gene expression (Johnson and Abramovitch 2017
6
Chinks in the Armor: Pathogenesis‐Based Strategies to Combat Bacterial Infections
Introduction
Virulence Factors: Pathogenesis‐Based Targets to Combat Bacterial Pathogens
Targeting Bacterial Host Cell Adhesion and Biofilm Formation
Quorum Sensing Inhibition
Interfering with Two‐Component Regulatory Systems
Stay updated, free articles. Join our Telegram channel
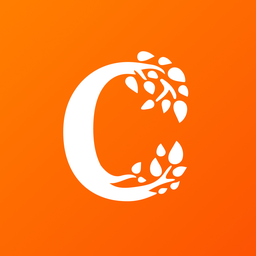
Full access? Get Clinical Tree
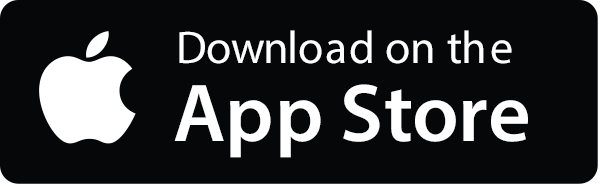
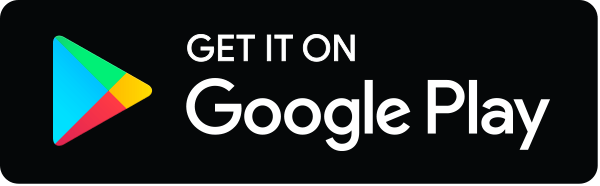